النبات
مواضيع عامة في علم النبات
الجذور - السيقان - الأوراق
النباتات الوعائية واللاوعائية
البذور (مغطاة البذور - عاريات البذور)
الطحالب
النباتات الطبية
الحيوان
مواضيع عامة في علم الحيوان
علم التشريح
التنوع الإحيائي
البايلوجيا الخلوية
الأحياء المجهرية
البكتيريا
الفطريات
الطفيليات
الفايروسات
علم الأمراض
الاورام
الامراض الوراثية
الامراض المناعية
الامراض المدارية
اضطرابات الدورة الدموية
مواضيع عامة في علم الامراض
الحشرات
التقانة الإحيائية
مواضيع عامة في التقانة الإحيائية
التقنية الحيوية المكروبية
التقنية الحيوية والميكروبات
الفعاليات الحيوية
وراثة الاحياء المجهرية
تصنيف الاحياء المجهرية
الاحياء المجهرية في الطبيعة
أيض الاجهاد
التقنية الحيوية والبيئة
التقنية الحيوية والطب
التقنية الحيوية والزراعة
التقنية الحيوية والصناعة
التقنية الحيوية والطاقة
البحار والطحالب الصغيرة
عزل البروتين
هندسة الجينات
التقنية الحياتية النانوية
مفاهيم التقنية الحيوية النانوية
التراكيب النانوية والمجاهر المستخدمة في رؤيتها
تصنيع وتخليق المواد النانوية
تطبيقات التقنية النانوية والحيوية النانوية
الرقائق والمتحسسات الحيوية
المصفوفات المجهرية وحاسوب الدنا
اللقاحات
البيئة والتلوث
علم الأجنة
اعضاء التكاثر وتشكل الاعراس
الاخصاب
التشطر
العصيبة وتشكل الجسيدات
تشكل اللواحق الجنينية
تكون المعيدة وظهور الطبقات الجنينية
مقدمة لعلم الاجنة
الأحياء الجزيئي
مواضيع عامة في الاحياء الجزيئي
علم وظائف الأعضاء
الغدد
مواضيع عامة في الغدد
الغدد الصم و هرموناتها
الجسم تحت السريري
الغدة النخامية
الغدة الكظرية
الغدة التناسلية
الغدة الدرقية والجار الدرقية
الغدة البنكرياسية
الغدة الصنوبرية
مواضيع عامة في علم وظائف الاعضاء
الخلية الحيوانية
الجهاز العصبي
أعضاء الحس
الجهاز العضلي
السوائل الجسمية
الجهاز الدوري والليمف
الجهاز التنفسي
الجهاز الهضمي
الجهاز البولي
المضادات الحيوية
مواضيع عامة في المضادات الحيوية
مضادات البكتيريا
مضادات الفطريات
مضادات الطفيليات
مضادات الفايروسات
علم الخلية
الوراثة
الأحياء العامة
المناعة
التحليلات المرضية
الكيمياء الحيوية
مواضيع متنوعة أخرى
الانزيمات
Patterns of Inheritance
المؤلف:
Cavenee, Webster K., and Raymond L. White
المصدر:
The Genetic Basis of Cancer
الجزء والصفحة:
27-10-2015
3110
Patterns of Inheritance
Whether an organism is a worm or a human, virtually all its characteristics are influenced by its genetic makeup. Since Gregor Mendel’s pioneering studies of inheritance in the mid-nineteenth century, enormous strides have been made in understanding the molecular basis of inheritance. With the blossoming of the biotechnology industry in the 1980s and the birth of the field of genomics in the 1990s, which seeks to sequence and study the entire genetic content of organisms, countless genes have been identified and their contributions to specific characteristics elucidated. Such understanding will amplify in the decades to come, undoubtedly leading to advances in many fields, but particularly in agriculture and medicine. With the draft of the human genome DNA sequence completed in 2001, scientists can anticipate a vast increase in their understanding of the molecular genetics of human disease.
Phenotype and Genotype
The phenotype includes all observable characteristics of an individual. Although Mendel’s studies were restricted to the outward traits of pea plants, such as flower color and plant height, phenotype can include characteristics observable only under certain circumstances or with specialized tools and technology. For example, a human phenotype certainly includes eye and skin color, but it also includes characteristics such as blood type and bone density.
A genotype is the complete genetic makeup of an individual. Nonetheless, as it has been impractical to consider the entire genetic makeup of an individual, the reference to genotype is usually restricted to those genes influencing the aspect of phenotype being studied at the time. In other words, if scientists are interested in studying the coat color trait in Labrador retrievers, they focus their attention on the gene(s) identified with influencing coat color.
A young native of the Solomon Islands. A slight variation in the activity of an enzyme for pigment synthesis may result in phenotypic variation
Proteins Dictate Phenotype
Genotype controls phenotype because genes direct the production of proteins. Proteins, in turn, dictate virtually every reaction in the cell and thus are directly responsible for observable characteristics.
How do the proteins work to direct phenotype? Some proteins serve structural functions (for example, in the maintenance of cell and/or tissue shape and rigidity) while others are involved in the transport of molecules and communication between cells. A substantial proportion of proteins are enzymes, catalyzing chemical reactions for the synthesis and transformation of virtually all biological molecules. The varying types and quantities of all biological molecules in the cells and tissues of an individual is what ultimately lead to phenotypic variation. For example, slight variation in the activity of an enzyme for pigment synthesis in a plant may result in white flowers rather than red. Likewise, a slight difference in a protein responsible for cell communication during the development of leaf tissue might result in variation of leaf shape. Understanding the path from genotype to phenotype is a major concern of modern molecular biology and one of the ultimate goals of the human genome project.
Mutation and Genetic Variation
If a particular gene is mutated (the deoxyribonucleic acid [DNA] sequence is somehow altered), the result can be a change in the amino acid sequence of a protein or the quantity of a protein produced. Such a change may affect phenotype for the organism in a detrimental manner (for example, a mutation that causes muscle deterioration in humans), a seemingly neutral manner (a change from purple to green stems in cultivated tomato plants), or sometimes even a beneficial manner (a mutation that allows a soil bacterium to survive freezing).
Mutation is an essential and ongoing component of evolution. All living organisms are “mutants” in some sense, having arisen by virtue of genetic change from an ancestor. Within a population, there may be many versions of a given gene, called alleles. The term “mutant” allele is used somewhat arbitrarily to describe a version of a gene that is found very rarely in the population, whereas any version of a gene is considered “wild-type” or “normal” if it is relatively common. Each human being contains a handful of mutated alleles because of errors in replication early in development, as well as a number of rare, harmful alleles each has inherited from his or her parents.
Mutation Affects Protein Function
In order to understand how mutations cause changes in phenotype, it is essential to study how mutations affect protein function. If one amino acid in the protein sequence is changed to another with very similar properties, the conformation of the folded protein may not be functionally altered. However, if the amino acid change is substantial (for instance, from small to large or from nonpolar to polar), the protein architecture may be altered in such a way as to cause a decline or abolition of function. It is likely that such a loss of function will ensue from mutation, since protein function has been fine-tuned during evolution and depends on the precise architecture of the protein. (If a person whacks a computer with a sledgehammer, it is unlikely that the computer’s performance will actually increase.) In some rare situations, however, instead of causing an ineffective protein, a mutation may result in a hyperactive protein. An enzyme may work at its job overtime, for example, by synthesizing excessive quantities of a product. Although a hardworking protein may sound like a benefit to the organism, this is rarely the case. Such gain-of-function mutations are usually toxic, disrupting the delicate balance of biomolecules needed for life.
Dominance Between Alleles
The path from allele to phenotype is complex in most organisms, since more than one allele of a given gene is usually present. In diploid organisms such as humans, an individual carries two alleles of each gene. If the individual carries two identical alleles (a homozygote), then the phenotype necessarily will reflect the only version present. However, if an individual carries two different alleles (a heterozygote), each encoding a slightly different characteristic, what will the phenotype show? For example, if a diploid plant carries one allele encoding red flowers and one allele encoding white flowers, will the flowers be red or white? The answer depends on the molecular behavior of the encoded proteins.
Imagine that the red flower allele encodes a functional enzyme essential for the synthesis of the chemical compound leading to red pigmentation. If the white flower allele is a loss-of-function mutation, the enzyme encoded by this allele will not be functional and consequently will not contribute toward the synthesis of red pigment; the absence of red pigment leads to white flowers. In the heterozygote, however, enough functional enzymes may be produced by one allele to result in pigmented flowers.
In this case, geneticists would describe the red allele as dominant and the white allele as recessive. If the allele encoding red flowers is dominant, then this allele will phenotypically mask expression of the recessive allele (in this case encoding white flowers), resulting in the expression of red flowers. Thus, in order for a recessive allele to be expressed in the phenotype, only the recessive alleles can be present.
Alternatives to Dominance
Two dissimilar alleles in a pair need not have a completely dominant/ recessive relationship. In fact, it is often the case that neither allele is fully dominant, causing the heterozygote to appear different from either homozygote. In one type of allelic relationship, termed incomplete or partial dominance, the heterozygote produces a phenotype that is intermediate between both homozygotes. Snapdragons provide a good example of partial dominance. In this organism, the two homozygotes produce either red or white flowers, but the heterozygote produces pink flowers. In this case, the single functional allele in the heterozygote does not produce enough enzymes to synthesize large quantities of red pigment; the result is just enough pigment to make the flowers appear pink.
In another type of allelic relationship, termed codominance, the heterozygote produces a phenotype that incorporates both phenotypes of the homozygotes. A codominant relationship between alleles is often more apparent at the cellular or molecular level. A good example of codominance is seen in human blood type. People who are homozygous for the A blood type allele produce an enzyme that adds a particular type of sugar onto the exterior of red blood cells, thereby producing the “A antigen.” Similarly, homozygotes for the B blood type allele produce a different type of sugar on red blood cells, leading to the presence of “B antigen.” Heterozygotes with one A and one B allele produce enzymes that deposit both different types of sugars, resulting in red blood cells displaying both the A and B antigens.
When Does Mutation Affect Phenotype in Diploids?
If a mutant allele is dominant, a heterozygous individual will display the mutant phenotype; if the mutant allele is recessive, heterozygotes will have a normal phenotype (although they will be “carriers” of the mutant allele). What determines whether a mutant allele is recessive or dominant?
Loss-of-function mutations are usually recessive because the single functional allele can often create adequate levels of protein. Such is the case with the recessive cystic fibrosis disease in humans. Most homozygous children with this lethal disorder do not live to adulthood, although advances in treatment have prolonged life significantly. The normal allele of the cystic fibrosis gene encodes a cell membrane protein that provides an essential function of transporting chloride ions across the cell membrane. Mutant alleles of the gene encode a defective protein, and consequently chloride transport is blocked in people who are homozygous for the defective allele. There are many downstream consequences to this defect that ultimately lead to the cystic fibrosis disease. The most serious effects are in the lungs and the pancreas. In heterozygous carriers of mutant alleles, however, the single normal allele produces enough functional protein to transport chloride effectively and these individuals lead healthy lives.
The most common mechanism by which a mutant allele is dominant is through a gain-of-function mutation. In one type of gain-of-function mutation, termed hypermorphic, the protein is produced in excessive quantities or is somehow hyperactive. The presence of a single normal allele can do nothing to tone down the activity resulting from the mutant allele. This situation is observed, for example, in an extreme type of dwarfism called achondroplasia and in many human cancers. In these cases, specific molecular switch proteins are continually in the “on” mode, causing a number of processes to occur when they otherwise would not.
In a second type of gain-of-function mutation, termed neomorphic, the mutant protein takes on a new, inappropriate role. Although the normal protein may function adequately in its usual role, it cannot stop the mutant protein from wreaking havoc in the cell. Such is the case with Huntington’s disease and some other human neurodegenerative disorders in which neurons in the brain slowly die off. The normal function of the protein responsible for Huntington’s disease has yet to be determined, but it is known that the mutant protein forms abnormal aggregates in neurons. Whether this, or some other less visible, function is the toxic event is not yet clear.
Living with a Single Allele
It is often the case that a loss-of-function mutation is fully recessive, allowing heterozygous human carriers of mutant alleles to lead completely healthy lives. But suppose a person has only a single allele of a gene. This is the normal situation for all human males, who carry only a single X chromosome along with a single Y chromosome (the sex determination chromosomes). The X is a large chromosome with many essential genes having functions outside of sex determination. Women have two X chromosomes and therefore have two alleles for all the genes that lie on the X.
Traits carried on the X or Y chromosome are known as “sex-linked traits.” Those on any of the other chromosomes are called “autosomal traits.”
While women can carry recessive alleles of X-linked genes without disease manifestation, males with a single recessive allele are forced to express it, as they lack the possibility to mask the effect with a dominant, normal allele. Affected males necessarily pass the mutant allele onto their daughters and cannot pass it onto their sons (because they give their sons a Y, not an X, chromosome).
Hemophilia is an example of a human disorder caused by a mutation of a gene on the X chromosome. Hemophilia is caused by a loss-of-function mutation in a gene encoding a blood-clotting protein. Before treatment was available, males often bled to death in childhood from a small cut.
Many Factors Influence Phenotype
Genetic studies clearly are facilitated by phenotypes readily identified as resulting from a single gene, inherited in a simple so-called Mendelian fashion (a one gene-one phenotype relationship). It is important to keep in mind, however, that genes do not work in isolation. The expression of a single gene is dependent on many other genes in the genome (the genetic background) and many external, or environmental, factors such as nutrition, climate, or exposure to infectious agents. Although the complete DNA
sequence analysis of a genome will hasten one’s understanding of the many genes responsible for a trait, the identification of environmental factors will undoubtedly be slower because understanding these factors requires careful and controlled experimentation and observation. Such conditions are difficult to study, particularly in humans.
The effect of environment is evident in many experimental organisms, such as fungi, where “conditional” mutants are artificially constructed and utilized in genetic studies. For instance, temperature-sensitive mutants grow well at certain temperatures but die at a slightly elevated temperature that normal cells can easily tolerate. Even in humans, some environmental contributions to the manifestation of disease have been identified. Until the molecular mechanism for the phenylketonuria disease (PKU) was elucidated, it was not known that the mental retardation normally associated with this disease could be prevented by diet. Homozygotes with this recessive condition are unable to metabolize the amino acid phenylalanine (also found in some artificial sweeteners). The resulting excessive buildup of phenylalanine leads to impairment of mental abilities. By placing homozygous-recessive babies on a diet restrictive of phenylalanine, normal brain function occurs during development.
Epistasis: Many Genes, One Effect
The contributions of more than one gene on phenotypic expression have been well documented in genetic studies. In many instances, one gene has been found to mask the expression of a second gene; the former gene is said to be epistatic to the latter. Epistasis relationships are often observed in biochemical pathways, which employ numerous proteins in an assembly-line fashion. If enzyme A normally converts compound X into compound Y, and then enzyme B converts compound Y into red flower pigment, B can only affect the phenotype if a functional allele for enzyme A is first expressed. If enzyme A loses function and is unable to produce compound Y, the red pigment will not be produced irrespective of whichever gene B alleles might be present. This situation differs from dominance, as it involves two separate genes (and two separate proteins).
A scanning electron micrograph showing healthy, round red blood cells and a diseased sickle-shaped cell.
Nonpenetrance
The influence of other, unidentified factors can be seen when some individuals in a study do not display the phenotype normally associated with their defined genotype, a situation termed nonpenetrance. For example, polydactyly is a dominant human disorder resulting in the development of more than five digits on hands and feet. Since the mutant allele is dominant, all individuals carrying the allele should display the phenotype. Nonetheless, some individuals have normal numbers of fingers and toes in spite of the presence of the dominant allele. Thus, there must be other factors that influence the penetrance of the mutant allele; these factors might be other genes in the individual’s genome, or certain unknown factors in the individual’s environment.
Pleiotropy: One Gene, Many Effects
Sickle cell disease is a very common genetically inherited disorder that is often fatal in childhood; although some treatment is available, there is no cure to date. The disease is characterized by anemia, extreme pain and fatigue, heart failure, spleen enlargement, severe microbial infection, and impaired mental abilities. Early genetic studies demonstrated that the disease is inherited in an autosomal (not linked to the sex chromosomes), recessive manner.
The responsible allele of sickle cell disease expresses a mutated version of a protein subunit of hemoglobin. Normal hemoglobin is found in red blood cells and is essential for the transport of oxygen to all tissues of the body. In sickle cell disease, the conformation of the mutant hemoglobin subunit is altered, by virtue of a single amino acid change from the wild-type subunit, causing the proteins to associate with each other into abnormal fibers. This association inhibits the binding of oxygen and causes the red blood cells to appear stretched out in a characteristic “sickle” shape. The sickle cells clog capillaries, seriously compounding the effect by reducing all blood flow to various tissues.
Sickle cell disease provides a useful example of how a highly complex phenotype can result from a single, well-defined genotype (in this case a single nucleotide mutation). Such a situation, termed pleiotropy, arises due to a cascade of consequences from the single mutated protein. In this case, the abnormal hemoglobin causes poor oxygen transport and sickling of red blood cells, which in turn causes anemia, poor blood circulation, and accumulation of sickle cells in the spleen. The poor blood circulation in turn causes heart failure, lung damage (often followed by pneumonia), and brain dam age. Overloading the spleen with sickle cells causes extreme pain and an increased frequency of infectious disease.
References
Cavenee, Webster K., and Raymond L. White. “The Genetic Basis of Cancer.” Scientific American 272, no. 3 (1995): 72-79.
Edwards, D. D. “Sudden Death Tied to Sickle-Cell Trait.” Science News 132 (1987): 197.
Fairbanks, Daniel J., and W. Ralph Andersen. Genetics: The Continuity of Life. Pacific Grove, CA: Brooks/Cole Publishing Company, 1999.
Griffiths, Anthony J. F., et al. Modern Genetic Analysis. New York: W. H. Freeman and Company, 1999.
Keefe, Richard S. E., and Philip D. Harvey. Understanding Schizophrenia: A Guide to the New Research on Causes and Treatment. New York: Free Press, 1994.
Lunkes, A., Y. Trottier, and J. L. Mandel. “Pathological Mechanisms in Huntington’s Disease and Other Polyglutamine Expansion Diseases.” Essays in Biochemistry 33 (1998): 149-163.
Nowak, Rachel. “Look Lively (Busy Lifestyle Could Delay Genetic Illness).” New Scientist 165, no. 2225 (2000): 10.
Online Mendelian Inheritance in Man, OMIM™. McKusick-Nathans Institute for Genetic Medicine, Johns Hopkins University and National Center for Biotechnology Information, National Library of Medicine, 2000. <http://www.ncbi.nim.nih. gov/omim/>.
Strachan, Tom, and Andrew P. Read. Human Molecular Genetics, 2nd ed. New York: Wiley-Liss, 1999.
Welsh, Michael J., and Alan E. Smith. “Cystic Fibrosis.” Scientific American 273, no. 6 (1995): 52-61.
الاكثر قراءة في الوراثة
اخر الاخبار
اخبار العتبة العباسية المقدسة
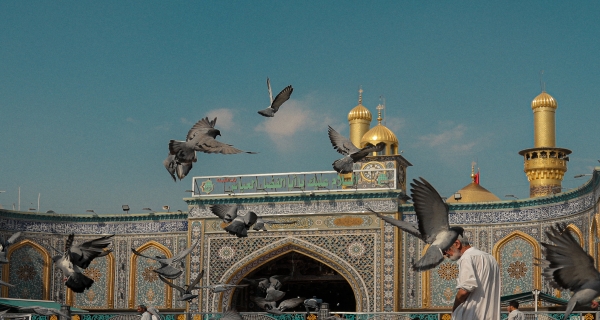
الآخبار الصحية
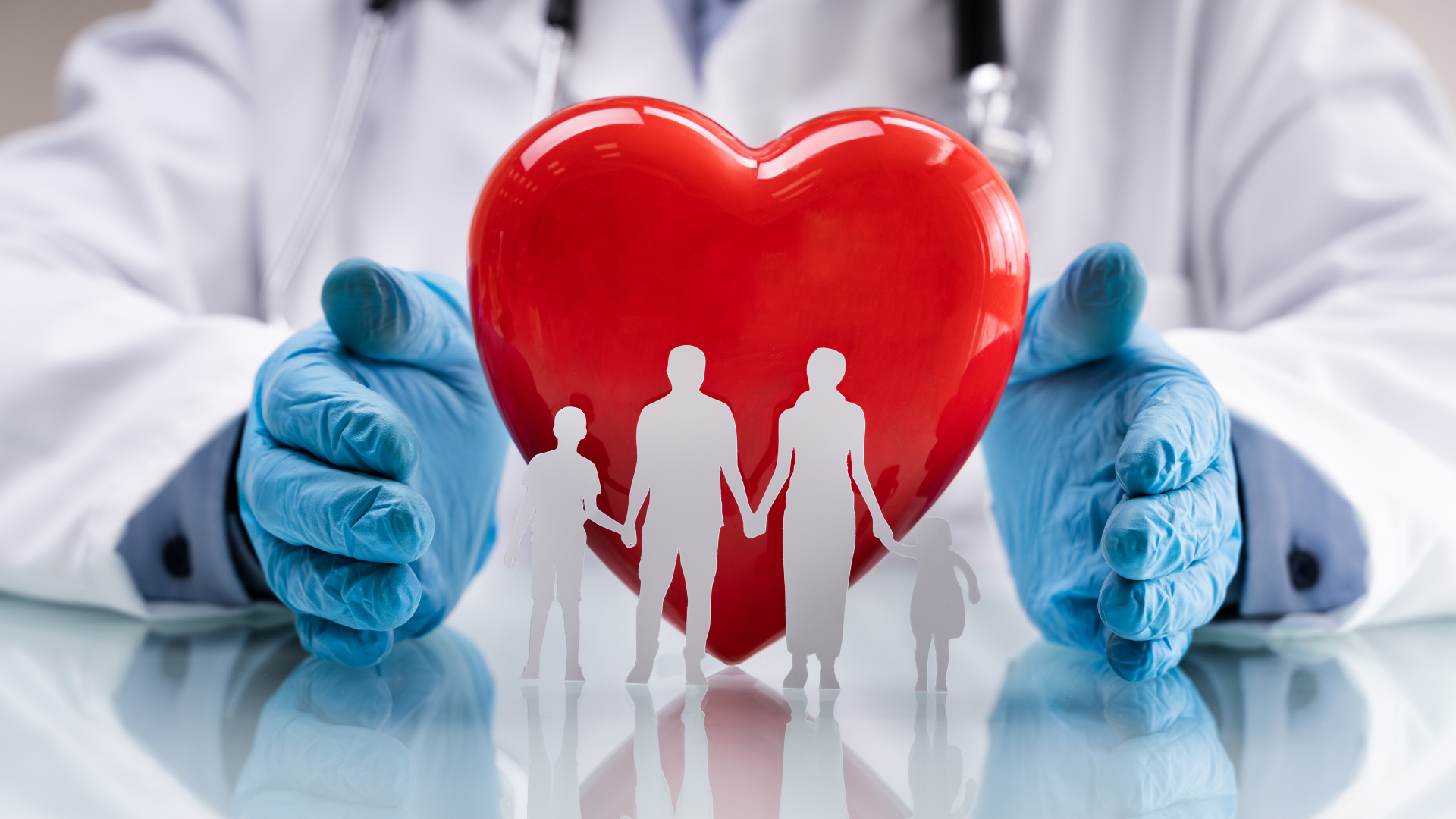