تاريخ الفيزياء
علماء الفيزياء
الفيزياء الكلاسيكية
الميكانيك
الديناميكا الحرارية
الكهربائية والمغناطيسية
الكهربائية
المغناطيسية
الكهرومغناطيسية
علم البصريات
تاريخ علم البصريات
الضوء
مواضيع عامة في علم البصريات
الصوت
الفيزياء الحديثة
النظرية النسبية
النظرية النسبية الخاصة
النظرية النسبية العامة
مواضيع عامة في النظرية النسبية
ميكانيكا الكم
الفيزياء الذرية
الفيزياء الجزيئية
الفيزياء النووية
مواضيع عامة في الفيزياء النووية
النشاط الاشعاعي
فيزياء الحالة الصلبة
الموصلات
أشباه الموصلات
العوازل
مواضيع عامة في الفيزياء الصلبة
فيزياء الجوامد
الليزر
أنواع الليزر
بعض تطبيقات الليزر
مواضيع عامة في الليزر
علم الفلك
تاريخ وعلماء علم الفلك
الثقوب السوداء
المجموعة الشمسية
الشمس
كوكب عطارد
كوكب الزهرة
كوكب الأرض
كوكب المريخ
كوكب المشتري
كوكب زحل
كوكب أورانوس
كوكب نبتون
كوكب بلوتو
القمر
كواكب ومواضيع اخرى
مواضيع عامة في علم الفلك
النجوم
البلازما
الألكترونيات
خواص المادة
الطاقة البديلة
الطاقة الشمسية
مواضيع عامة في الطاقة البديلة
المد والجزر
فيزياء الجسيمات
الفيزياء والعلوم الأخرى
الفيزياء الكيميائية
الفيزياء الرياضية
الفيزياء الحيوية
الفيزياء العامة
مواضيع عامة في الفيزياء
تجارب فيزيائية
مصطلحات وتعاريف فيزيائية
وحدات القياس الفيزيائية
طرائف الفيزياء
مواضيع اخرى
Baryon Number Violation
المؤلف:
Leonard Susskind And James Lindesay
المصدر:
AN INTRODUCTION TO BLACK HOLES, INFORMATION, AND THE STRING THEORY REVOLUTION
الجزء والصفحة:
16-12-2015
1820
Baryon Number Violation
The conservation of baryon number is the basis for the incredible stability of ordinary matter. Nevertheless, there are powerful reasons to believe that baryon number, unlike electric charge, can at best be an approximate conservation law. The obvious difference between baryon number and electric charge is that baryon number is not the source of a long range gauge field. Thus it can disappear without some flux having to suddenly change at infinity.
Consider a typical black hole of stellar mass. It is formed by the collapse of roughly 1057 nucleons. Its Schwarzschild radius is about 1 kilometer, and its temperature is 10−8 electron volts. It is far too cold to emit anything but very low energy photons and gravitons. As it radiates, its temperature increases, and at some point it is hot enough to emit massive neutrinos and anti-neutrinos, then electrons, muons, and pions. None of these carry off baryon number. It can only begin to radiate baryons when its temperature has increased to about 1 GeV. Using the connection between mass, the mass of the black hole at this point is about 1010 kilograms. This is a tiny fraction of the original black hole mass, and even if it were to decay into nothing but protons, it could produce only about 1037 of them. Baryon number must be violated by quantum gravitational effects.
In fact, most modern theories predict baryon violation by ordinary quantum field theoretic processes. As a simplified example, let's suppose there is a heavy scalar particle X which can mediate a transition between an elementary proton and a prositron, as well as between two positrons, as in Figure 1.1. Since the X-boson is described by a real field, it cannot carry any quantum numbers, and the transition evidently violates baryon conservation. The proton could then decay into a positron and an electron positron pair. Let's also assume that the coupling has the usual Yukawa form
where g is a dimensionless coupling.
Fig. 1.1. Feynman diagrams of p→ Xe+ and e+ → Xe+.
If the mass MX is sufficiently large, baryon conservation will be a very good symmetry at ordinary energies, just as it is in the real world. The proton will have a lifetime in excess of 1032 years.
Now let us consider what happens when a proton falls into a black hole. The baryon number is lost, and will not be radiated back out in the Hawking radiation. The question is: where does the baryon violation take place? One possible answer is that it occurs when the freely falling proton encounters very large curvature invariants as the singularity is approached. From the proton's viewpoint, there is nothing that would stimulate it to decay before that.
On the other hand, from the vantage point of the external observer, the proton encounters enormously high temperatures as it approaches the horizon. Temperatures higher than MX can certainly excite the proton to decay. So the external observer will conclude that baryon violation can take place at the horizon. Who is right?
The answer that black hole complementarity implies is that they are both right. On the face of it, that sounds absurd. Surely the event of proton decay takes place in some definite place. For a very large black hole, the time along the proton trajectory between horizon crossing and the singularity can be enormous. Thus, it is difficult to understand how there can be an ambiguity.
The real proton propagating through space-time is not the simple structureless bare proton. The interactions cause it to make virtual transitions from the bare proton to a state with an X-boson and a positron. The complicated history of the proton is described by Feynman diagrams such as shown in Figure 1.2. The diagrams make evident that the real proton is
Fig. 1.2. Proton virtual fluctuation into X e+ pair.
a superposition of states with different baryon number; in the particular processes shown in Figure 1.2, the intermediate state has vanishing baryon number.
Nothing about virtual baryon non-conservation is especially surprising. As long as the X-boson is sufficiently massive, the rate for real proton decay will be very small, and the proton will be stable for long times.
What is surprising is that the probability to find the proton in a configuration with vanishing baryon number is not small. This probability is closely related to the wave function renormalization constant of the proton, and is of the order
where κ is the cutoff in the field theory. For example, for g ∼ 1, κ of the order of the Planck mass, and MX of the order 1016 GeV, the probability that the proton has the “wrong” baryon number is of order unity. This might seem paradoxical, since the proton is so stable.
The resolution of the paradox is that the proton is continuously making extremely rapid transitions between baryon number states. The transitions take place on a time scale of order δt ∼ 1/MX. Ordinary observations of the proton do not see these very rapid fluctuations. The quantity that we normally call baryon number is really the time averaged baryon number normalized to unity for the proton.
Now let us consider a proton passing through a horizon, as shown in Figure 1.3. Since the probability that the proton is actually an X, e+ system is not small, it is not unlikely that when it passes the horizon, its instantaneous baryon number is zero. But it is clear from Figure 1.3 that from the viewpoint of an external observer, this is not a short-lived intermediate state. A fluctuation that is much too rapid to be seen by a low energy observer falling with the proton appears to be a real proton decay lasting to eternity from outside the horizon.
Fig. 1.3. Proton fluctuations while falling through horizon.
This, of course, is nothing but the usual time dilation near the horizon. As a proton or any other system approaches the horizon, internal oscillations or fluctuations appear to indefinitely slow down, so that a short-lived virtual fluctuation becomes stretched out into a real process.
The process of baryon violation near the horizon should not be totally surprising to the external observer. To him, the proton is falling into a region of increasing temperature. At a proper distance M−1X from the horizon, the temperature becomes of order MX. Baryon violating processes are expected at that temperature.
An interesting question is whether an observer falling with the proton can observe the baryon number just before crossing the horizon, and send a message to the outside world that the proton has not decayed. The answer is interesting. In order to make an observation while the proton is in a region of temperature >MX the observer must do so very quickly. In the proton's frame, the time spent in the hot region before crossing the horizon is 1/MX. Thus, to obtain a single bit of information about the state of the proton, the observer has to probe it with at least one quantum with energy of order MX. But such an interaction between the proton and the probe quantum is at high enough energy that it can cause a baryon violating interaction even from the perspective of the proton's frame of reference. Thus the observer cannot measure and report the absence of baryon violation at the horizon without himself causing it.
It is evident from this example that the key to understanding the enormous discrepancies in the complementary description of events lies in understanding the fluctuations of matter at very high frequencies; frequencies so high that the ordinary low energy observer has no chance of detecting them.
الاكثر قراءة في الثقوب السوداء
اخر الاخبار
اخبار العتبة العباسية المقدسة
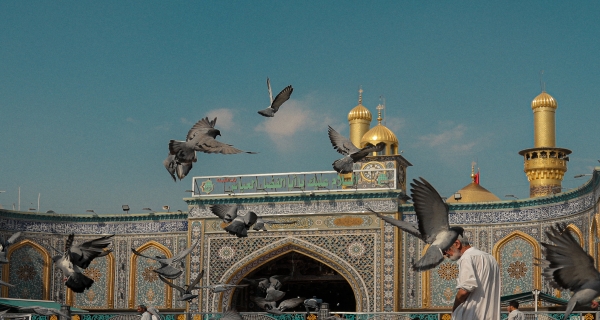
الآخبار الصحية
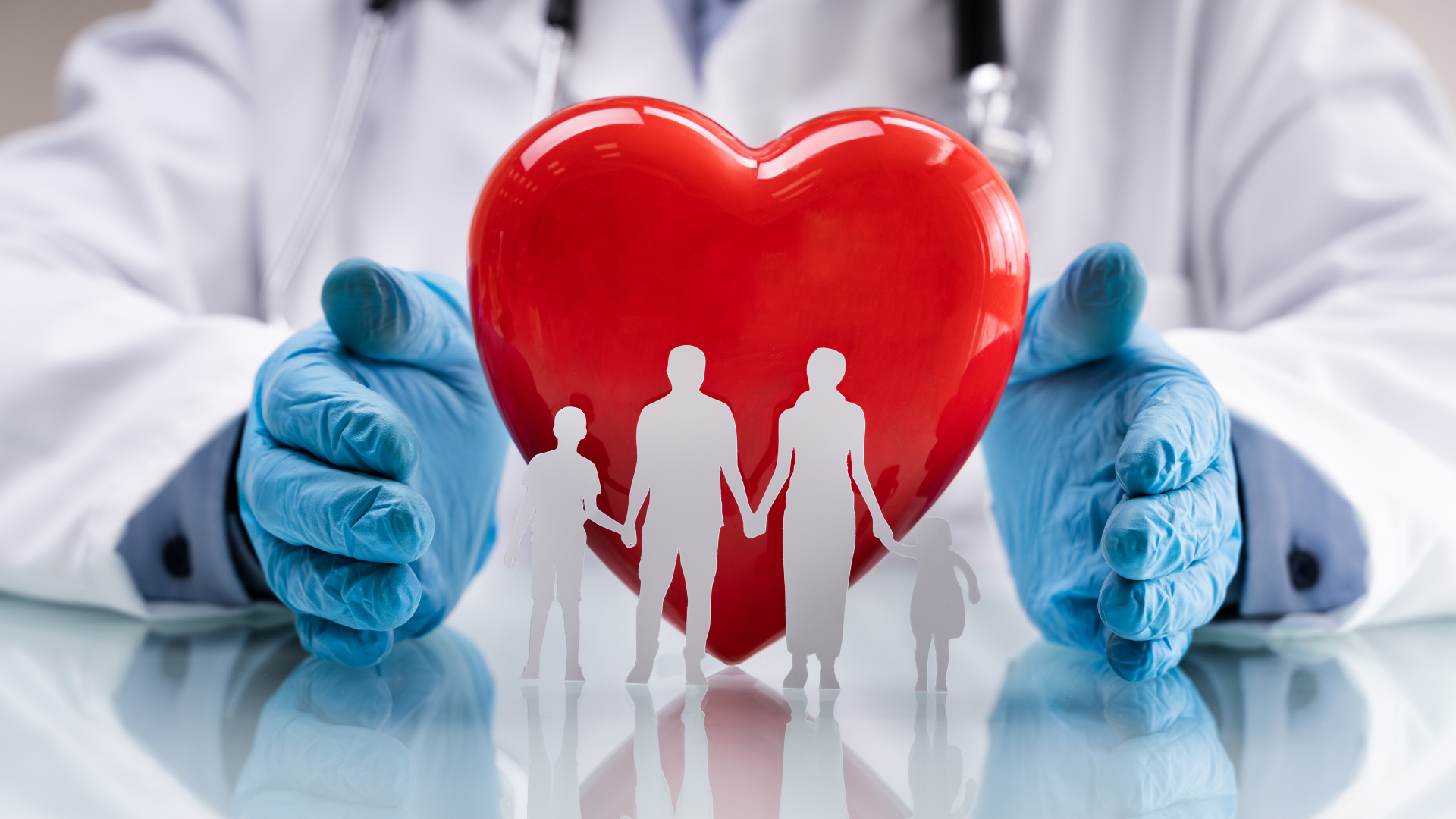