النبات
مواضيع عامة في علم النبات
الجذور - السيقان - الأوراق
النباتات الوعائية واللاوعائية
البذور (مغطاة البذور - عاريات البذور)
الطحالب
النباتات الطبية
الحيوان
مواضيع عامة في علم الحيوان
علم التشريح
التنوع الإحيائي
البايلوجيا الخلوية
الأحياء المجهرية
البكتيريا
الفطريات
الطفيليات
الفايروسات
علم الأمراض
الاورام
الامراض الوراثية
الامراض المناعية
الامراض المدارية
اضطرابات الدورة الدموية
مواضيع عامة في علم الامراض
الحشرات
التقانة الإحيائية
مواضيع عامة في التقانة الإحيائية
التقنية الحيوية المكروبية
التقنية الحيوية والميكروبات
الفعاليات الحيوية
وراثة الاحياء المجهرية
تصنيف الاحياء المجهرية
الاحياء المجهرية في الطبيعة
أيض الاجهاد
التقنية الحيوية والبيئة
التقنية الحيوية والطب
التقنية الحيوية والزراعة
التقنية الحيوية والصناعة
التقنية الحيوية والطاقة
البحار والطحالب الصغيرة
عزل البروتين
هندسة الجينات
التقنية الحياتية النانوية
مفاهيم التقنية الحيوية النانوية
التراكيب النانوية والمجاهر المستخدمة في رؤيتها
تصنيع وتخليق المواد النانوية
تطبيقات التقنية النانوية والحيوية النانوية
الرقائق والمتحسسات الحيوية
المصفوفات المجهرية وحاسوب الدنا
اللقاحات
البيئة والتلوث
علم الأجنة
اعضاء التكاثر وتشكل الاعراس
الاخصاب
التشطر
العصيبة وتشكل الجسيدات
تشكل اللواحق الجنينية
تكون المعيدة وظهور الطبقات الجنينية
مقدمة لعلم الاجنة
الأحياء الجزيئي
مواضيع عامة في الاحياء الجزيئي
علم وظائف الأعضاء
الغدد
مواضيع عامة في الغدد
الغدد الصم و هرموناتها
الجسم تحت السريري
الغدة النخامية
الغدة الكظرية
الغدة التناسلية
الغدة الدرقية والجار الدرقية
الغدة البنكرياسية
الغدة الصنوبرية
مواضيع عامة في علم وظائف الاعضاء
الخلية الحيوانية
الجهاز العصبي
أعضاء الحس
الجهاز العضلي
السوائل الجسمية
الجهاز الدوري والليمف
الجهاز التنفسي
الجهاز الهضمي
الجهاز البولي
المضادات الحيوية
مواضيع عامة في المضادات الحيوية
مضادات البكتيريا
مضادات الفطريات
مضادات الطفيليات
مضادات الفايروسات
علم الخلية
الوراثة
الأحياء العامة
المناعة
التحليلات المرضية
الكيمياء الحيوية
مواضيع متنوعة أخرى
الانزيمات
Deoxyribonucleotide Biosynthesis And Degradation
المؤلف:
B. A. Kunz, S. E. Kohalmi, T. A. Kunkel, C. K. Mathews, E. M. McIntosh, and J. A. Reidy
المصدر:
Mutation Res. 318, 1–64
الجزء والصفحة:
1-1-2016
4735
Deoxyribonucleotide Biosynthesis And Degradation
Most cells contain five to ten times as much RNA as DNA. The deoxyribonucleotide precursors to DNA use the same pathways for synthesis of purine and pyrimidine rings as do the ribonucleotides that are used for RNA synthesis and for a host of other metabolic functions. Therefore, the pathways that lead from the de novo purine and pyrimidine synthetic pathways toward deoxyribonucleoside triphosphates (dNTPs) are quantitatively minor. They are of obvious significance, however. Not only must they be sufficiently active to supply the cell's needs for DNA replication, recombination, and DNA repair but they must be activated and deactivated in coordination with the timing of DNA replication during the cell cycle because dNTPs have no known metabolic roles other than their function as DNA precursors. Moreover, the pathways must be regulated so as to provide the four DNA precursors at rates corresponding to the base composition of the genome being served. dNTP pool imbalances that develop when regulatory mechanisms are perturbed have numerous biological consequences (1)—including mutagenesis, recombination, induction of latent viruses, and cell death—caused by stimulation of apoptosis (2).
1. Pathways to dNTPs
1.1. Chemical Differences between RNA and DNA
It is useful to begin our survey of deoxyribonucleotide biosynthesis by considering the processes through which the chemical differences between DNA nucleotides and RNA nucleotides arise. The methyl group of thymine, which distinguishes it from uracil, arises through the transfer of a single-carbon functional group to a uracil nucleotide, catalyzed by thymidylate synthase. The presence of 2-deoxyribose as the sugar in DNA nucleotides rather than the ribose found in RNA comes about through reduction of the ribose sugar on a ribonucleotide substrate. Some aerobic bacteria and all anaerobic microorganisms studied carry out this reduction at the ribonucleoside triphosphate (rNTP) level. In all other organisms studied, however, the substrates for ribonucleotide reductase are the ribonucleoside 5′-diphosphates (rNDPs). Whether a particular reductase acts on rNDPs or rNTPs, a single enzyme reduces all four ribonucleotide substrates. Accordingly, the enzyme interacts with allosteric modifiers to ensure that the four DNA precursors are produced at rates commensurate with the base composition of the organism's genome. In this article, we will describe the predominant pathways to dNTPs that begin with reduction of rNDPs to deoxyribonucleoside diphosphates (dNDPs). These pathways are summarized in Figure 1.
Figure 1. Pathways of dNTP biosynthesis de novo. Although these pathways are widespread, they are not universal, as indicated in the text. Enzyme 1, ribonucleoside diphosphate reductase; 2, nucleoside diphosphate kinase; 3, dCMP kinase (probably); 4, dCMP deaminase; 5, dUTPase; 6, thymidylate synthase; 7, thymidylate kinase. CH2 = THF is 5,10-methylenetetrahydrofolate, and DHF is dihydrofolate.
1.2. The Role of Nucleoside Diphosphate Kinase
For three of the four nucleotides—ADP, CDP, and GDP—the conversion to the dNTP involves simply a phosphorylation of the rNDP reductase product, catalyzed by nucleoside diphosphate kinase. In most cells, this enzyme is very active, and all known forms of the enzyme have very low specificity. Thus, the enzyme catalyzes the reversible transfer of the g-phosphate of any common rNTP or dNTP to the phosphate at the b-position of any common rNDP or dNDP. The equilibrium constant for each reaction catalyzed is close to unity. Thus, the direction in which a nucleoside diphosphate kinase-catalyzed reaction occurs in vivo depends on the concentrations of substrates and products. Because ATP is almost always the most abundant intracellular nucleoside triphosphate, most such reactions involve the ATP-dependent conversion of a ribo- or deoxyribonucleoside diphosphate to the corresponding triphosphate.
1.3. Thymine Nucleotide Biosynthesis
The biosynthesis of thymidine triphosphate is obviously more complex than that of any other dNTP given that thymine nucleotides are derived from uracil nucleotides and a methyl group must be created. In most cells, the dUDP produced by rNDP reductase action on UDP is phosphorylated to dUTP by nucleoside diphosphate kinase. dUTP is cleaved by an active pyrophosphatase, deoxyuridine triphosphatase, or dUTPase. This enzyme plays a dual role: (1) Because dUTP is a good substrate for DNA polymerase, dUTPase minimizes the steady-state pool size of dUTP and, hence, helps to exclude dUMP from DNA. (2) The dUTPase reaction is a significant biosynthetic route to dUMP, the substrate for thymidylate synthase.
Thymidylate synthase catalyzes the transfer of a single-carbon functional group from 5,10-methylenetetrahydrofolate to position 5 of the pyrimidine ring in deoxyuridine monophosphate, yielding thymidine monophosphate (Fig. 2). dTMP is phosphorylated to dTDP by , and conversion to dTTP involves nucleoside diphosphate kinase.
Figure 2. The thymidylate synthesis cycle. Enzyme 1, thymidylate synthase; 2, dihydrofolate reductase; 3, serine transhydroxymethylase. dR-P is deoxyribose 5′-phosphate; PABA is p-aminobenzoate; Glun refers to the multiple glutamate residues on naturally occurring folate coenzymes.
In the thymidylate synthase reaction, the transferred methylene group must be reduced to the methyl level, and the electron pair that brings this reduction about comes from the reduced pteridine ring of 5,10-methylenetetrahydrofolate. The coenzyme, therefore, loses both its methylene group and an electron pair, leading to dihydrofolate. Transformation of the coenzyme for reuse involves, first, its reduction to tetrahydrofolate by dihydrofolate reductase and, next, transfer of a single-carbon group to the pteridine ring, usually catalyzed by serine transhydroxymethylase. The stoichiometric requirement for the folate cofactor in the thymidylate synthase reaction probably explains the selective toxicity of dihydrofolate reductase inhibitors toward proliferating cells (3). Such inhibitors include Methotrexate, widely used in cancer chemotherapy, and Trimethoprim, an antibacterial agent that specifically inhibits dihydrofolate reductases of prokaryotic origin(see Aminopterin). Proliferating cells have a continuous requirement for dTTP synthesis, to sustain DNA replication. The greater the flux rate through thymidylate synthase in vivo, the more rapidly tetrahydrofolate pools will be depleted after administration of a dihydrofolate reductase inhibitor and, hence, the greater will be the sensitivity of those cells toward the growth-inhibiting or lethal effects of blockage of dihydrofolate reductase.
1.4. Biosynthetic Routes to dUMP
As noted earlier, the cleavage of deoxyuridine triphosphate to the corresponding monophosphate, catalyzed by dUTPase, provides a significant source of dUMP for the synthesis of thymine nucleotides. In most cells, however, an additional route, involving deamination of deoxycytidine nucleotides, is more important in a quantitative sense. As shown in Figure 3, the hydrolytic deamination in most organisms involves conversion of dCMP to dUMP, catalyzed by dCMP deaminase. In a few bacteria, including Escherichia coli, the deamination occurs at the triphosphate level, with conversion of dCTP to dUTP, catalyzed by dCTP deaminase. In such organisms, the conversion of CDP to dUMP occurs by the following pathway:
Figure 3. dCMP deaminase as a branch point between dCTP and dTTP synthesis. Allosteric modifiers of dCMP deaminase are shown, as is the regulation of salvage synthetic pathways. Enzyme 1, ribonucleoside diphosphate reductase; 2, nucleoside diphosphate kinase; 3, dCMP kinase (possibly); 4, dUTPase; 5, dCMP deaminase; 6, dCMP kinase; 7, thymidylate synthase; 8, thymidylate kinase; 9, deoxycytidine kinase; 10, thymidine kinase.
The most widespread pathway, shown in Figure 3, involves deamination at the monophosphate level. In many vertebrate cell lines, this is the predominant, often exclusive, pathway leading to dUMP (4). It is not clear why the in vivo flux rate from UDP to dUDP is so low, particularly when the activities of ribonucleotide reductase on CDP and UDP are regulated virtually identically as shown by assays of the purified enzyme in vitro (5). Whatever the reason, dCMP deaminase is an important metabolic branch point between routes to dTTP and dCTP. Allosteric regulation of this enzyme—activation by dCTP and inhibition by dTTP—ensures that these two dNTPs are produced at relative rates commensurate with their need for DNA synthesis. dCMP deaminase is not essential for cell viability, at least as determined in cell culture systems. However, mutant cells lacking dCMP deaminase have abnormally high dCTP pools (1, 6), and the resultant increase in the [dCTP]/[dTTP] pool ratio often brings about a mutator phenotype, in which the dCTP pool expansion stimulates its incorporation opposite template nucleotides other than dGMP (1).
2. Deoxyuridine Nucleotide Metabolism
As noted earlier, dUTPase plays a role in providing dUMP for the thymidylate synthase reaction. Of equal or greater importance is its role in minimizing the incorporation of dUMP into DNA in place of dTMP. Cells possess two independent mechanisms to minimize the amount of dUMP in DNA: (1) dUTPase acts to deplete the dUTP pool, and hence to minimize dUMP incorporation by DNA polymerase, usually opposite dAMP in template DNA. (2( The second mechanism is a base excision repair process, starting with . This enzyme scans DNA, and when it contacts a dUMP residue, it cleaves the glycosidic bond, probably by a base-flipping mechanism (7). This reaction creates an abasic site, which initiates a repair process that results in insertion of the correct nucleotide at that site (8).
Because the base-pairing properties of uracil and thymine are essentially identical, it seems likely that the mechanisms for uracil exclusion from DNA are not designed primarily to replace dUMP with dTMP, when that dUMP is base-paired with dAMP in the template DNA. Instead, the probable target for DNA uracil exclusion is dUMP residues incorrectly paired with dGMP, but DNA-uracil N-glycosylase simply recognizes every dUMP residue in DNA, no matter what its partner. G · U DNA base pairs would arise most frequently by deamination of a dCMP residue paired in duplex DNA with dGMP. Cytosine undergoes spontaneous deamination at appreciable rates whether as a free nucleic acid precursor or as a residue in DNA or RNA. If C in a G · C base pair underwent deamination, the resultant G · C base pair would generate a G · C and an A · U base pair in the next round of replication and, ultimately, the deamination would lead to a G · C → A · T transition mutation. Thus, the DNA-uracil exclusion systems probably act to maintain stability of the genome.
3. Salvage Routes to dNTPs
As noted in Salvage pathways to nucleotide biosynthesis, deoxyribonucleotide salvage pathways involving uptake of extracellular precursors primarily use deoxyribonucleoside kinases. Human cells contain four such enzymes of varying specificities, two located in the cytosol and two in mitochondria, whereas other organisms, such as E. coli, contain thymidine kinase as the only deoxyribonucleoside kinase. Thymidine kinase has received particularly intensive study, partly because of the mechanism of its cell cycle regulation (9) but largely because the enzyme is so useful as a means for incorporating radiolabel into DNA. For reasons still not clear, thymidine competes extremely effectively with the de novo synthetic pathway to dTTP such that, in many animal cell systems, radiolabeled thymidine is incorporated into DNA at full specific activity, often bypassing substantial endogenous pools generated by de novo synthesis (10). One popular experimental organism for which this does not work is yeast; fungi lack thymidine kinase. Investigators have circumvented this difficulty, however, by designing yeast strains that are permeable to dTMP, strains for which exogenous dTMP can be used as a labeled DNA precursor.
For salvage of deoxyribonucleoside monophosphates released by intracellular DNA degradation, the deoxyribonucleoside monophosphate kinases play the key roles. Animal cells contain four such enzymes, each specific for one deoxyribonucleotide, ie, dAMP kinase, dCMP kinase, dGMP kinase, and dTMP kinase . The enzyme phosphorylating dAMP acts also on AMP and is the well-known adenylate kinase, or myokinase. dCMP kinase acts also on UMP, and dTMP kinase acts also on dUMP. dTMP kinase is involved also in de novo dTTP synthesis, as shown in Figure 1. dCMP kinase may also play a role in de novo dNTP synthesis. The enzyme converting dCDP (produced by ribonucleotide reductase) to dCMP, en route to dUMP and dTMP, has still not been identified. Since nucleotide kinases all have equilibrium constants close to 1, it is quite possible that the role of dCMP kinase is to carry out the synthesis of dCMP:
By contrast, dAMP and dGMP kinases play roles only in nucleotide salvage reactions because the de novo pathways lead directly from ribonucleoside diphosphate to deoxyribonucleoside diphosphate to deoxyribonucleoside triphosphate.
The action of nucleoside phosphorylases provides another route to salvage dNTP synthesis, eg, deoxyadenosine + Pi → adenine + deoxyribose-1-phosphate. Now the deoxyribose-1-phosphate can react with another base in a nucleoside phosphorylase-catalyzed reaction proceeding in the reverse direction, eg, dR-1-P + Thy → dThd + Pi. Thymidine can then become a nucleotide via the thymidine kinase reaction leading to dTMP. Although this process can significantly change relative dNTP pool sizes, it does not involve net deoxyribonucleotide synthesis; rather, it involves redistribution of the deoxyribosyl units linked to purine and pyrimidine bases.
4. Biosynthesis of Novel Nucleotides in Bacteriophage Infection
Much of what is known about pathways of dNTP synthesis in bacterial and animal cells came from investigations of the biosynthesis of unusual modified nucleotides in infection by certain bacteriophage (11). T-even bacteriophages of E. coli contain 5-hydroxymethylcytosine completely substituted for cytosine; many hydroxymethyldeoxycytidylate (HM-dCMP) residues in phage DNA are further modified by glycosidic links through the hydroxymethyl group to one or two glucose residues. These modifications occur through the action of phage-encoded enzymes that catalyze reactions comparable to those in cellular metabolism (Fig. 4). For example, the hydroxymethylation reaction is carried out by an enzyme, dCMP hydroxymethylase, that transfers a single-carbon group from methylenetetrahydrofolate to C-5 of dCMP, much as thymidylate synthase modifies C-5 of the pyrimidine dUMP (12); in fact, T-even phages encode a thymidylate synthase that displays significant amino acid sequence homology with dCMP hydroxymethylase. Not shown in Figure 4 is the involvement of glucosylation in the transfer of glucose to hydroxymethyl groups of HM-dCMP residues after their incorporation into DNA.
Figure 4. Metabolic pathways induced in bacteriophage infection. Solid arrows identify reactions known to be catalyzed by phage-coded enzymes; dashed arrows identify reactions catalyzed only by enzymes encoded by the host cell genome.
Other modifications are carried out by bacteriophage SP01 and SP82 of Bacillus subtilis, in which 5-hydroxymethyluracil substitutes for thymine and two other B. subtilis phages, PBS1 and PBS2, which replace thymine with uracil. The relevant pathways are also shown in Figure 4. Finally, the figure shows pathways induced in Xanthomonas oryzae by XP12, a bacteriophage in which cytosine is completely replaced by 5-methylcytosine. These base modifications help the virus to parasitize the host bacterium, overrunning pathways used by the host cell to produce its own DNA. Perhaps it should not be surprising that these are all virulent phages.
5. Deoxyribonucleoside Catabolism
5.1. Substrate Cycles and dNTP Pool Regulation
Nucleotide kinase-catalyzed reactions are readily reversible. Whereas these enzymes have long been considered to participate primarily in dNTP synthesis, there is now good evidence that nucleotide kinases function in both directions in vivo and that they can participate in dNTP degradation (13). For example, when DNA replication was blocked in mammalian cells with aphidicolin, intracellular dNTPs were degraded, and deoxyribonucleosides were excreted into the medium in significant amounts (13, 14), with little change in the steady-state pool size of the four dNTPs. The implication of these findings is that dNTP pool sizes are controlled not only at the level of synthesis but are also regulated by catabolism.
Under conditions that do not interfere with DNA synthesis in cultured cells, pulse-chase experiments show that the rate of utilization of a particular dNTP for DNA synthesis is equivalent to the rate at which the pool of that dNTP turns over (15, 16). These observations argue against the compartmentation of dNTP pools in animal cells; with one known exception, all the dNTP molecules in these cells are accessible to the replication machinery. The one exception is dCTP labeled by deoxycytidine (13); much of this pool is used for synthesis of the deoxycytidine counterparts to cytidine diphosphate-containing lipid precursors, such as CDP-choline. It is not yet clear whether these dCDP-containing “liponucleotides” play a metabolic role distinct from those of the more abundant cytidine-containing lipids.
For cells that don't excrete deoxyribonucleosides but instead degrade them further, that further degradation usually involves attack by nucleoside phosphorylases to yield deoxyribose-1-phosphate plus the free base. Further degradation of the purine and pyrimidine bases proceeds by pathways outlined in Purine ribonucleotide metabolism and Pyrimidine ribonucleotide metabolism.
5.2. Enzymatic Degradation of dGTP
A widely distributed enzyme is a dGTP pyrophosphatase , which catalyzes the hydrolytic cleavage of dGTP to dGMP and pyrophosphate. The metabolic role of this enzyme was obscure until it was found in E. coli that the enzyme is encoded by the mutT gene (17). The mutT gene product acts to prevent transversion mutations that proceed by G · C → T · A and A · T → C · G pathways. Mutant alleles of mutT cause spontaneous rates of these transversion mutations at other loci to increase by 100- to 10,000-fold. Mutations of these types are caused by oxidizing agents, such as hydrogen peroxide, which act in large part by oxidizing guanine residues in DNA to 8-oxoguanine. This oxidized purine readily base-pairs with adenine, setting in motion the events leading to mutagenesis. These realizations led to the discovery that the MutT protein is far more active in cleaving 8-oxo-dGTP than dGTP. Thus, it seems clear that the mutagenic effects of oxidizing agents include oxidation of guanine, not as a DNA residue but as a DNA precursor that is incorporated into DNA and then stimulates a transversion pathway by pairing with adenine. The MutT nucleotidase prevents this by degrading the mutagenic DNA substrate, 8-oxo-dGTP, before it has a chance to be incorporated into DNA.
E. coli contains a second dGTPase, which is distinctive in that it hydrolyzes dGTP to deoxyguanosine plus tripolyphosphate. Unlike the MutT dGTPase, which is widely distributed in organisms, this other dGTPase is found only in enteric bacteria (18). Its metabolic role is still obscure.
References
1. B. A. Kunz, S. E. Kohalmi, T. A. Kunkel, C. K. Mathews, E. M. McIntosh, and J. A. Reidy (1994) Mutation Res. 318, 1–64.
2. F. J. Oliver, M. K. L. Collins, and A. López-Rivas (1996) Biochem. J. 316, 421–425.
3. J. J. McCormack (1981) Med. Res. Revs. 1, 303–331.
4. V. Bianchi, E. Pontis, and P. Reichard (1985) Mol. Cell. Biol. 7, 4218–4224.
5.L. Thelander and P. Reichard (1979) Ann. Rev. Biochem. 48, 133–158.
6. G. L. Weinberg, B. Ullman, and D. W. Martin Jr. (1981) Proc. Natl. Acad. Sci. USA 78, 2447–2451.
7. R. J. Roberts (1995) Cell 82, 9–12.
8. E. C. Friedberg, G. C. Walker, and W. Siede (1995) DNA Repair and Mutagenesis, ASM Press, Washington DC, pp. 147–153.
9. J. L. Sherley and T. J. Kelly (1988) J. Biol. Chem. 263, 8350–8358.
10. C. K. Mathews (1975) Exptl. Cell Res. 92, 47–56.
11. C. K. Mathews (1977) In Comprehensive Virology, Vol. 7, (H. Fraenkel-Conrat and R. R. Wagner, eds.), Plenum Press, New York, pp. 179–294.
12. S. S. Cohen (1968) Virus-Induced Enzymes. Columbia Univesity Press, New York.
13. P. Reichard (1988) Ann. Rev. Biochem. 57, 349–374.
14. B. Nicander and P. Reichard (1985) J. Biol. Chem. 260, 9216–9222.
15. B. Nicander and P. Reichard (1983) Proc. Natl. Acad. Sci. USA 80, 1347–1351.
16. V. Bianchi, E. Pontis, and P. Reichard (1992) Exptl. Cell Res. 199, 120–128.
17. H. Maki and M. Sekiguchi (1992) Nature 355, 273–275.
18. S. Quirk and M. J. Bessman (1991) J. Bacteriol. 173, 6665–6669.
الاكثر قراءة في مواضيع عامة في الاحياء الجزيئي
اخر الاخبار
اخبار العتبة العباسية المقدسة
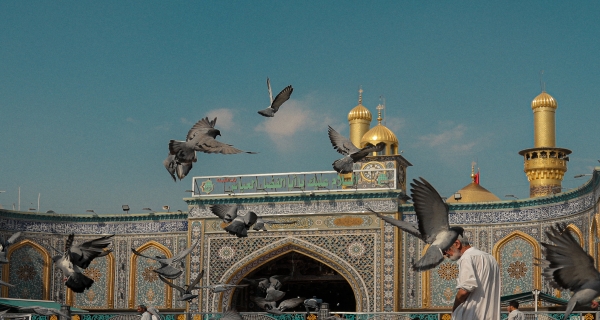
الآخبار الصحية
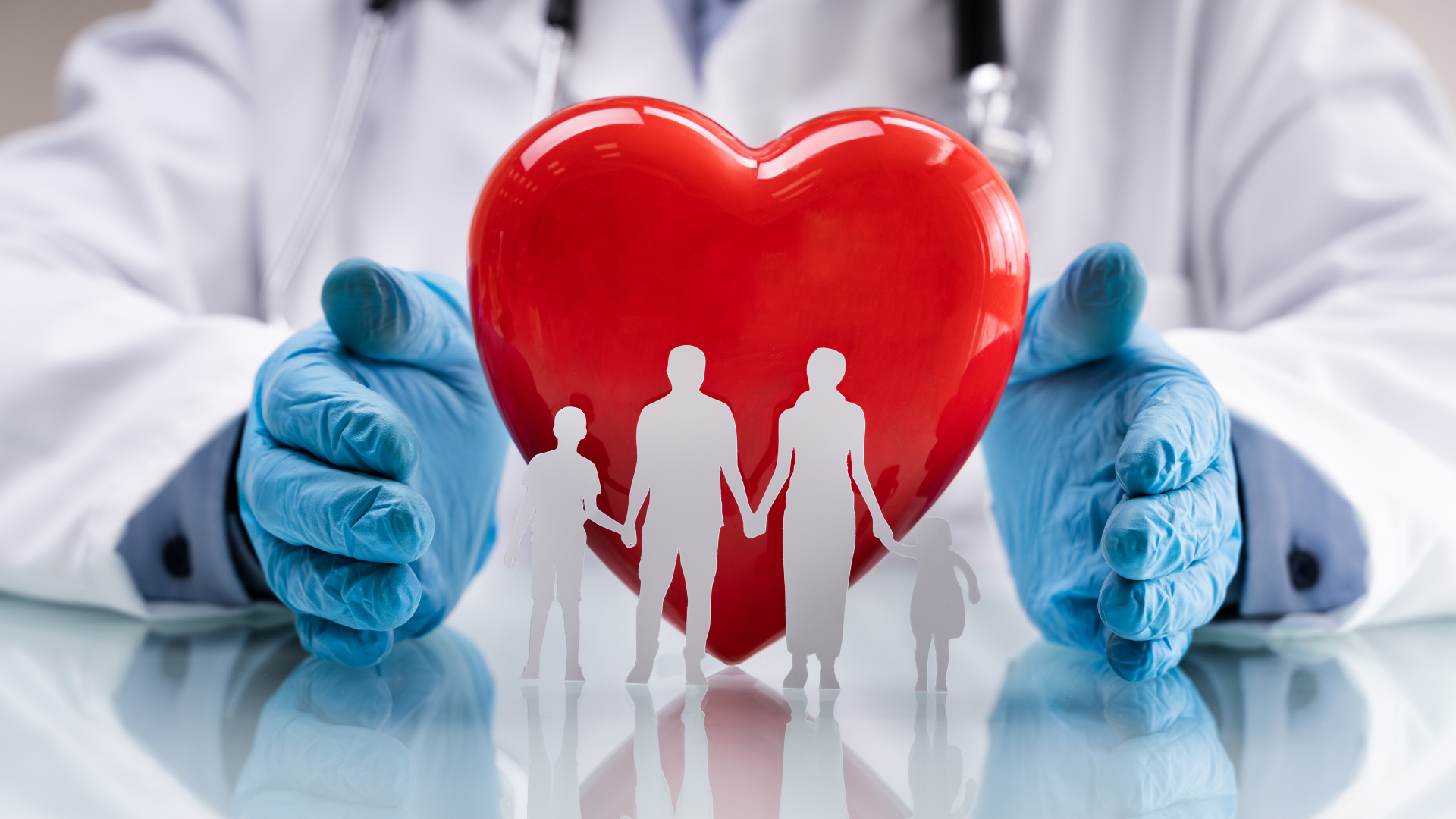