النبات
مواضيع عامة في علم النبات
الجذور - السيقان - الأوراق
النباتات الوعائية واللاوعائية
البذور (مغطاة البذور - عاريات البذور)
الطحالب
النباتات الطبية
الحيوان
مواضيع عامة في علم الحيوان
علم التشريح
التنوع الإحيائي
البايلوجيا الخلوية
الأحياء المجهرية
البكتيريا
الفطريات
الطفيليات
الفايروسات
علم الأمراض
الاورام
الامراض الوراثية
الامراض المناعية
الامراض المدارية
اضطرابات الدورة الدموية
مواضيع عامة في علم الامراض
الحشرات
التقانة الإحيائية
مواضيع عامة في التقانة الإحيائية
التقنية الحيوية المكروبية
التقنية الحيوية والميكروبات
الفعاليات الحيوية
وراثة الاحياء المجهرية
تصنيف الاحياء المجهرية
الاحياء المجهرية في الطبيعة
أيض الاجهاد
التقنية الحيوية والبيئة
التقنية الحيوية والطب
التقنية الحيوية والزراعة
التقنية الحيوية والصناعة
التقنية الحيوية والطاقة
البحار والطحالب الصغيرة
عزل البروتين
هندسة الجينات
التقنية الحياتية النانوية
مفاهيم التقنية الحيوية النانوية
التراكيب النانوية والمجاهر المستخدمة في رؤيتها
تصنيع وتخليق المواد النانوية
تطبيقات التقنية النانوية والحيوية النانوية
الرقائق والمتحسسات الحيوية
المصفوفات المجهرية وحاسوب الدنا
اللقاحات
البيئة والتلوث
علم الأجنة
اعضاء التكاثر وتشكل الاعراس
الاخصاب
التشطر
العصيبة وتشكل الجسيدات
تشكل اللواحق الجنينية
تكون المعيدة وظهور الطبقات الجنينية
مقدمة لعلم الاجنة
الأحياء الجزيئي
مواضيع عامة في الاحياء الجزيئي
علم وظائف الأعضاء
الغدد
مواضيع عامة في الغدد
الغدد الصم و هرموناتها
الجسم تحت السريري
الغدة النخامية
الغدة الكظرية
الغدة التناسلية
الغدة الدرقية والجار الدرقية
الغدة البنكرياسية
الغدة الصنوبرية
مواضيع عامة في علم وظائف الاعضاء
الخلية الحيوانية
الجهاز العصبي
أعضاء الحس
الجهاز العضلي
السوائل الجسمية
الجهاز الدوري والليمف
الجهاز التنفسي
الجهاز الهضمي
الجهاز البولي
المضادات الحيوية
مواضيع عامة في المضادات الحيوية
مضادات البكتيريا
مضادات الفطريات
مضادات الطفيليات
مضادات الفايروسات
علم الخلية
الوراثة
الأحياء العامة
المناعة
التحليلات المرضية
الكيمياء الحيوية
مواضيع متنوعة أخرى
الانزيمات
Monohybrid Crosses
المؤلف:
AN INTRODUCTION TO PLANT BIOLOGY-1998
المصدر:
JAMES D. MAUSETH
الجزء والصفحة:
19-10-2016
2776
Monohybrid Crosses
Sexual reproduction between two individuals is called a cross. The meiotic divisions that precede a cross reduce the number of sets of chromosomes per cell from the diploid number to the haploid number. Consequently, each sex cell—that is, each sperm cell and egg cell—contains one complete set of genes. Furthermore, each sperm cell contains all the genes necessary to construct a new plant; the same is true of each egg cell. The zygote (the fertilized egg) has two complete sets of genes.
Within a population, mutations produce new alleles, and the genotypes of individuals within the population differ. Of the plants that grow in an area and that can interact sexually, many may have the same allele of a particular gene but other individuals may have other alleles, other versions of the gene. Consequently, the alleles carried by a particular sperm cell may or may not be identical to the homologous alleles of the egg it fertilizes.
MONOHYBRID CROSSES WITH INCOMPLETE DOMINANCE
In a monohybrid cross only a single character is analyzed and studied; the inheritance of other traits is not considered. For instance, a plant with red flowers might be crossed with one that produces white flowers, and only the inheritance of that flower color trait is studied. Characters involving flower shape, leaf structure, and photosynthetic efficiency are also being inherited simultaneously, but in a monohybrid cross, only one is studied. This makes the analysis and understanding of the results much simpler. Once basic principles of a particular trait are known, then its interaction with another factor (dihybrid cross) or two other factors (trihybrid cross) can be studied.
Consider the cross just mentioned: A plant with red flowers is bred to one with white flowers. It does not matter which flower produces pollen and which produces ovules. Once the cross is made, seeds and fruit develop; the seeds are planted, and when mature, the new plants are allowed to flower so that their flower color can be examined. The flowers of all plants in this new generation are pink, resembling each parent somewhat, but not exactly like either (Fig. 1). The parents are called the parental generation, the offspring of their crossbreeding are the F1 or first filial generation, and if these interbreed, their offspring are the F2 generation.
FIGURE 1: (a) A monohybrid cross analyzing the trait of flower color. Details are explained in the text. (b) The phenotypes of parents, gametes, and progeny.
The molecular biology of this monohybrid, flower color cross is easy to understand. Each parent is diploid and thus has two copies of the gene involved. In the red-flowered parent, both alleles produce mRNA that is translated into functional enzymes involved in the synthesis of red pigment. In a white-flowered parent, both alleles are defective. It may be that each produces an mRNA that when translated results in a protein unable to perform the necessary reaction. Or the promoter region may be mutated and can no longer interact with a chemical messenger. Whatever the cause, there is no pigment, and the flower white.
Using genetic symbols, the red-flowered parent is RR and the white-flowered one is rr (Fig. 1). Each parent is said to be homozygous, because each has two identical alleles for this gene. The pink-flowered F1 has received an R allele from one parent and an r allele from the other, so its genotype is Rr; it is heterozygous because it has two different alleles for this gene. We use these symbols even though we have neither isolated the gene nor analyzed its nucleotide sequence; R and r are simply labels. Most genes are known only by their phenotypes and the labels given to them by geneticists (Table 16.2). With a genotype of Rr, the plant produces mRNA, half of which carries the defect; thus only half the normal amount of enzyme is produced. This results in less pigment being formed, only enough to make the flower look pink, not red. Neither parental trait dominates the other, so this pair of alleles shows incomplete dominance: The heterozygous phenotype differs from both homozygous phenotypes.
When analyzing the possible outcomes of crosses and breeding, one must understand the types and quantities of gametes involved. All the plants we are considering are diploid and form haploid spores by meiosis. The RR parent has chromosomes as shown in Figure 16.10b, and regardless of how chromosomes separate during meiosis, all spores receive an R allele. In the rr parent, all spores receive an r allele. Because the RR parent produces only R spores, it also produces only R sex cells, both sperms and eggs in typical bisexual flowers. Similarly, the rr parent produces, indirectly, only r gametes. When the two plants are interbred, an R gamete unites with an r gamete, establishing a heterozygous (Rr) zygote that grows into a heterozygous adult by means of mitotic cell divisions. No other outcome is possible; it does not matter which gamete is which, an R sperm and an r egg result in the same type of zygote as an r sperm and an R egg.
When the heterozygote matures and flowers, each spore mother cell produces two types of spores (Fig. 2), not just one as is true of a homozygote. Because each cell has one R allele and one r allele, during the first meiotic division, one daughter cell receives R and the other receives r. The second division of meiosis results in two R spores and two r spores. If this is occurring in the anther, half the pollen grains and hence half the sperms have R, and the other half have r. In the nucellus of many plants, only one megaspore survives. If this gene has no effect on spore metabolism (and a gene for flower color is probably inactive in spores), then half the time R cells survive and half the time r cells live. In heterozygote parents, the two types of sperms and eggs are produced in equal numbers.
FIGURE 2:Production of haploid cells by meiosis in a heterozygote. During anaphase I the patenal chromosome is separated from the maternal chromosome, so one type of allele is separated from the other. As sister chromatids separate during anaphase II, each daughter nudeus receives identical alleles.
CROSSING HETEROZYGOTES WITH THEMSELVES
When a plant's own pollen is used to fertilize its own eggs, the cross is a selfing. A plant can also be selfed by being crossed with another plant with exactly the same genotype. Selfing heterozygotes has interesting, instructive consequences; 50% (on average) of all sperms and eggs contain the R allele and 50% have r (Fig. 2). Not all zygotes are identical genotypically: Some are RR, having resulted from an R sperm and an R egg, others are rr (r sperm and r egg), and some are Rr (either R sperm and r egg or r sperm and R egg). Selfing a heterozygote produces three types of F1s, some of which (Rr) resemble the parents and others (RR and rr) the grandparents. Again, to analyze the results, we must wait for the zygote to develop into an embryo, then plant the seeds and wait until the new plants are old enough to flower. Because each genotype produces a distinct phenotype, the genotype of each plant is known simply by looking at the flowers. If a large number of heterozygotes (pink-flowered plants) are selfed and large numbers of Fi plants grown, about one fourth are red (RR), one half pink (Rr), and one fourth white (rr). This is an important ratio, typically represented as 1:2:1, and should be memorized immediately.
The reason for the proportion of these genotypes is explained in Figure 3. A Punnett square can be set up in which all types of one gamete, say, the egg, are arranged along the top of the square, and all types of the other gamete are arranged on the left side. The boxes are then filled in with the allele symbol above it and to the left. Because the gametes are produced in a ratio of 1 R: 1 r, listing them as in Figure 3 automatically represents their relative numbers in nature.
FIGURE 3 :A Punnett square makes it easy to analyze and understand the results of a cross. Gametes from one parent are listed across the top; those of the other parent are listed on the left side.
The 1:2:1 ratio was one of the great discoveries of Gregor Mendel (1822-1884), an Austrian monk who performed experiments with pea plants. His cross-breeding experiments became the basis for modern genetics. Mendel discovered that in a selfing of this type, the recovery of the parental types means that genetic material must be composed of particles, such that the R genetic material can be separated from the r genetic material in the Rr heterozygote. Prior to Mendel's work, it was thought that the genetic material was a fluid. Two fluids cannot mix and then separate again perfectly. The constancy of the 1:2:1 tatio made it more logical to think in terms of discrete particles, genes, that never lost their identity regardless of the crosses in which they participated.
Furthermore, the 1:2:1 ratio can be realistically interpreted only in terms of each individual plant having two copies—being diploid—and each sex cell having one copy —being haploid. If each plant had only one copy, pink-flowered heterozygotes would be impossible, whereas if each had three, phenotypes such as dark pink (RRr) and light pink (Rr) should also be present. Keep in mind the state of scientific knowledge in 1865 when Mendel was working. The concept that all organisms are composed of cells with nuclei had only recently been proposed; mitosis and cell division were very poorly understood, and meiosis would not be discovered for another 23 years. The concepts of chromosomes and homologous pairs would not be well established until the 20th century, and the existence of mRNA was not confirmed until the mid-1960s.
Mendel's work was well known but was years ahead of its time. Advances in microscopy and chromosome staining were necessary to recognize homologous chromosomes and the diploid nature of most plant and animal cells. But as soon as these were discovered, scientists immediately realized that chromosomes must be the carriers of Mendel's hypothetical genes.
Evolution by natural selection had just been discovered and was revolutionizing not only biology but also all aspects of philosophy, theology, anthropology, and cosmology— the very ways in which we think about our world. Natural selection required diversity and change, whereas Mendel's discoveries appeared to show that differences in phenotype were due only to the mixing and separating of unchanged, constant genes; early proponents of evolution were certain that Mendel's theory of genes as the basis of inheritance had to be incomplete. In 1927, mutations were proven to occur; genes are just changeable enough to be the basis of both evolution by natural selection and Mendelian genetics. With the discovery of mutations, the two monumental discoveries of the 1800s were reconciled.
MONOHYBRID CROSSES WITH COMPLETE DOMINANCE
The situation in which only half as much product of an enzyme, such as the red pigment discussed above, is produced in a heterozygote is not a universal situation . In certain species or with other traits, cytoplasmic control mechanisms may cause the enzyme to function until a specific amount of product is synthesized. The enzyme may have to work faster or longer, but the final amount of product is the same whether the plant has two functional alleles or only one. In other situations, the amount of enzyme might be monitored such that the nonmutant mRNA of the heterozygote is translated more frequently or rapidly.
In either case, the phenotype of the heterozygote is like that of the parent with two effective alleles. That trait is said to be dominant over the other version of the trait, which is recessive. An example is height. A tall plant with a TT genotype produces sex cells that are all T. A short plant, genotype tt, produces only t sex cells (Fig. 4). When the tall plant is crossed with the short one, all Fl progeny have the Tt genotype, but they all have the phenotype of being tall, indistinguishable from their TT, homozygous dominant parent. We do not know what protein is produced by the T allele, but even with only one functional allele, enough product is made to permit normal growth. Tt plants are tall, and the tall character completely dominates the "dwarf" character.
FIGURE 4:In this monohybrid cross, the trait "tall" shows complete dominance over the trait "dwarf." In a Tt nucleus, the T allele may be transcribed twice as much as each T allele in a TT nucleus, or the resulting mRNA may be translated twice as much, or the protein may work twice as long or twice as fast. Or a TT plant may contain a level of T protein far above the threshold for responsiveness.
Knowing the molecular biology of genetic systems, you can predict that when hetero- zygotes are selfed, two types of sperm cells (T and t) and two types of eggs (T and t) are produced. The Punnett square for the cross is as in Figure 5, and a genotype ratio of 1 TT. 2 Tt. 1 tt is expected. The prediction is correct, but what will the phenotype ratio be? one out of four plants will be tall due to a TT genotype, and two out of four will be tall due to a Tt genotype. Thus, three fourths have the tall phenotype and one fourth have the shot phenotype. Whenever a cross is made and a phenotype ratio of 3.1 is seen, we should suspect that the parents are heterozygous and the character shows complete dominance.
FIGURE 5:In setting up the Punnett square for a selling of Tt plants, first establish the genotypes of the two parents. Then determine what types of gametes are produced and in what proportions, and fill in the squares with the genotypes. From the genotypes, the phenotypes in each square can be determined.
TEST CROSSES
When traits with incomplete dominance are studied, the genotype of any plant is easy to determine from its phenotype. If the trait has complete dominance, it is difficult to know what the genotype of any particular plant is unless the plant shows the recessive trait. Imagine you are studying the inheritance of tallness; you have selfed some heterozygotes and planted the resulting seeds. In your greenhouse or garden, there are now hundreds of plants, approximately 75% of which are tall and 25% short. You know the short ones are tt, and if you need a plant with the tt genotype for experimentation, you know automatically to chose short plants for the experiment. But if you need to experiment on plants with the TT genotype, how can you tell which they are? A tall plant picked at random is more likely to be a Tt plant, because there are twice as many of them as TT tall plants.
The genotype can be revealed by a test cross, a cross involving the plant in question and one that is homozygous recessive for the trait being studied. All gametes produced by a homozygous recessive parent carry the recessive allele, which is unable to mask the homologous allele in the resulting Fi zygote. If the plant being tested is actually homozygous dominant (TT), 100% of the progeny will be heterozygous (Tt) and tall (Fig. 6). If the tall parent is heterozygous, half its progeny in the test cross will be tall (Tt) and half short (tt). If only a few seeds resulting from the test cross are grown, it is possible statistically to choose all of them, by accident, from the TT group; but if a large number of seeds are grown, the chance of accidentally not choosing any of the Tt seeds is very low, and if even one of the F1 plants is short, the test parent must have been Tt.
FIGURE 6: Test crosses to determine if a plant with the dominant phenotype is heterozygous or homozygous. Of the data in this diagram, you would not know the genotypes of the test parents before the test cross; those data are what you are trying to discover. If you want to find only a single homozygote or heterozygote, you would need to do test crosses on only one or a few plants. But imagine that you had done an experimental cross for a trait that you assumed would give you a 3 : 1 ratio; it would be necessary to do test crosses on a large number of progeny plants with the dominant phenotype just to confirm your experiment .
When test crosses must be made on annual plants, the results are usually not known until after the plants have died. Their genotypes are then known, but the plants cannot be used for experimentation or breeding. In such cases, it usually is necessary to do both test crosses and experimental crosses simultaneously, not knowing which plants have the correct genotype for the experiment being performed. After the test cross results are complete, the parents that had the proper genotype can be identified and the experimental crosses that involved them can be analyzed.
MULTIPLE ALLELES
Each gene may have many alleles, not just two as in the examples discussed so far (7and t, R and r). A protein of average size consists of about 300 amino acids, so the coding portion of its mRNA must have about 300 codons, each containing three nucleotides. The gene is therefore at least 900 nucleotides long, not counting introns and promoters. At least 900 sites exist at which point mutations can occur, and of course any mutation may involve several nucleotides. Consequently, the gene may exist in many forms, called multiple alleles . When genes are polymorphic, having multiple alleles, numbers, such as Xi, X2, X3, X4, and so on, are used rather than capital and lower-case letters. Certain mutations still result in the production of a protein with the normal sequence, but most lead to altered protein structure. Some of these proteins are quite similar to the onginal protein, perhaps having similar or even identical enzymatic activity. However, many carry out the proper reaction more slowly or are not accurately controlled by regulatory mechanisms in the cytoplasm; a normal, wild-type phenotype may not be produced. With multiple alleles, the concept of dominance is more complex; one allele may produce the proper quantities of the functional protein, whereas a different allele produces more, another produces less, and a fourth produces one with altered activity. Many different phenotypes are possible.
Within a population of plants, as many types of gametes can be produced as there are different types of alleles. A heterozygous X1X2 plant can be crossed with a heterozygous XX one, resulting in progeny such as in Figure 16.17: X1X3, X1X4, X2X3, and X2X4. Four distinct types of F1 plant are produced, none of which has the genotype of either parent.
الاكثر قراءة في مواضيع عامة في علم النبات
اخر الاخبار
اخبار العتبة العباسية المقدسة
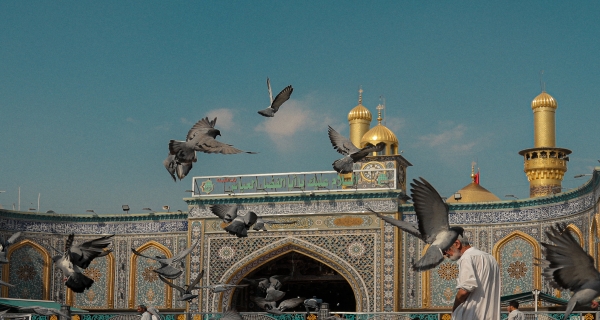
الآخبار الصحية
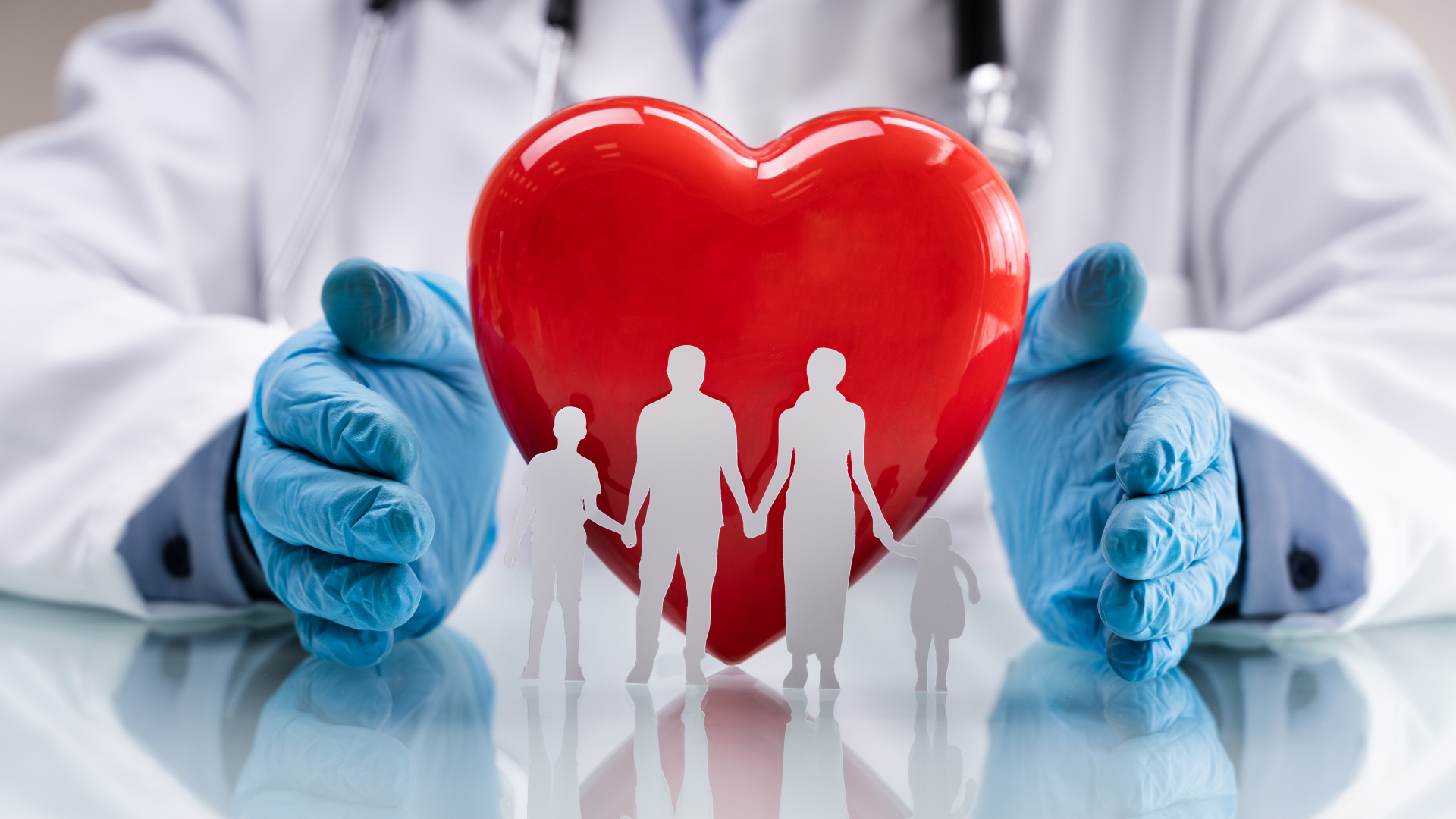