تاريخ الفيزياء
علماء الفيزياء
الفيزياء الكلاسيكية
الميكانيك
الديناميكا الحرارية
الكهربائية والمغناطيسية
الكهربائية
المغناطيسية
الكهرومغناطيسية
علم البصريات
تاريخ علم البصريات
الضوء
مواضيع عامة في علم البصريات
الصوت
الفيزياء الحديثة
النظرية النسبية
النظرية النسبية الخاصة
النظرية النسبية العامة
مواضيع عامة في النظرية النسبية
ميكانيكا الكم
الفيزياء الذرية
الفيزياء الجزيئية
الفيزياء النووية
مواضيع عامة في الفيزياء النووية
النشاط الاشعاعي
فيزياء الحالة الصلبة
الموصلات
أشباه الموصلات
العوازل
مواضيع عامة في الفيزياء الصلبة
فيزياء الجوامد
الليزر
أنواع الليزر
بعض تطبيقات الليزر
مواضيع عامة في الليزر
علم الفلك
تاريخ وعلماء علم الفلك
الثقوب السوداء
المجموعة الشمسية
الشمس
كوكب عطارد
كوكب الزهرة
كوكب الأرض
كوكب المريخ
كوكب المشتري
كوكب زحل
كوكب أورانوس
كوكب نبتون
كوكب بلوتو
القمر
كواكب ومواضيع اخرى
مواضيع عامة في علم الفلك
النجوم
البلازما
الألكترونيات
خواص المادة
الطاقة البديلة
الطاقة الشمسية
مواضيع عامة في الطاقة البديلة
المد والجزر
فيزياء الجسيمات
الفيزياء والعلوم الأخرى
الفيزياء الكيميائية
الفيزياء الرياضية
الفيزياء الحيوية
الفيزياء العامة
مواضيع عامة في الفيزياء
تجارب فيزيائية
مصطلحات وتعاريف فيزيائية
وحدات القياس الفيزيائية
طرائف الفيزياء
مواضيع اخرى
Birefringence
المؤلف:
Richard Feynman, Robert Leighton and Matthew Sands
المصدر:
The Feynman Lectures on Physics
الجزء والصفحة:
Volume I, Chapter 33
2024-03-24
1229
Another interesting effect of polarization is the fact that there are substances for which the index of refraction is different for light linearly polarized in one direction and linearly polarized in another. Suppose that we had some material which consisted of long, nonspherical molecules, longer than they are wide, and suppose that these molecules were arranged in the substance with their long axes parallel. Then what happens when the oscillating electric field passes through this substance? Suppose that because of the structure of the molecule, the electrons in the substance respond more easily to oscillations in the direction parallel to the axes of the molecules than they would respond if the electric field tries to push them at right angles to the molecular axis. In this way we expect a different response for polarization in one direction than for polarization at right angles to that direction. Let us call the direction of the axes of the molecules the optic axis. When the polarization is in the direction of the optic axis the index of refraction is different than it would be if the direction of polarization were at right angles to it. Such a substance is called birefringent. It has two refrangibilities, i.e., two indexes of refraction, depending on the direction of the polarization inside the substance. What kind of a substance can be birefringent? In a birefringent substance there must be a certain amount of lining up, for one reason or another, of unsymmetrical molecules. Certainly a cubic crystal, which has the symmetry of a cube, cannot be birefringent. But long needlelike crystals undoubtedly contain molecules that are asymmetric, and one observes this effect very easily.
Let us see what effects we would expect if we were to shine polarized light through a plate of a birefringent substance. If the polarization is parallel to the optic axis, the light will go through with one velocity; if the polarization is perpendicular to the axis, the light is transmitted with a different velocity. An interesting situation arises when, say, light is linearly polarized at 45∘ to the optic axis. Now the 45∘ polarization, we have already noticed, can be represented as a superposition of the x- and the y-polarizations of equal amplitude and in phase, as shown in Fig. 33–2(a). Since the x- and y-polarizations travel with different velocities, their phases change at a different rate as the light passes through the substance. So, although at the start the x- and y-vibrations are in phase, inside the material the phase difference between x- and y-vibrations is proportional to the depth in the substance. As the light proceeds through the material the polarization changes as shown in the series of diagrams in Fig. 33–2. If the thickness of the plate is just right to introduce a 90∘ phase shift between the x- and y-polarizations, as in Fig. 33–2(c), the light will come out circularly polarized. Such a thickness is called a quarter-wave plate, because it introduces a quarter-cycle phase difference between the x- and the y-polarizations. If linearly polarized light is sent through two quarter-wave plates, it will come out plane-polarized again, but at right angles to the original direction, as we can see from Fig. 33–2(e).
One can easily illustrate this phenomenon with a piece of cellophane. Cellophane is made of long, fibrous molecules, and is not isotropic, since the fibers lie preferentially in a certain direction. To demonstrate birefringence, we need a beam of linearly polarized light, and we can obtain this conveniently by passing unpolarized light through a sheet of polaroid. Polaroid, which we will discuss later in more detail, has the useful property that it transmits light that is linearly polarized parallel to the axis of the polaroid with very little absorption, but light polarized in a direction perpendicular to the axis of the polaroid is strongly absorbed. When we pass unpolarized light through a sheet of polaroid, only that part of the unpolarized beam which is vibrating parallel to the axis of the polaroid gets through, so that the transmitted beam is linearly polarized. This same property of polaroid is also useful in detecting the direction of polarization of a linearly polarized beam, or in determining whether a beam is linearly polarized or not. One simply passes the beam of light through the polaroid sheet and rotates the polaroid in the plane normal to the beam. If the beam is linearly polarized, it will not be transmitted through the sheet when the axis of the polaroid is normal to the direction of polarization. The transmitted beam is only slightly attenuated when the axis of the polaroid sheet is rotated through 90∘. If the transmitted intensity is independent of the orientation of the polaroid, the beam is not linearly polarized.
Fig. 33–3. An experimental demonstration of the birefringence of cellophane. The electric vectors in the light are indicated by the dotted lines. The pass axes of the polaroid sheets and optic axes of the cellophane are indicated by arrows. The incident beam is unpolarized.
To demonstrate the birefringence of cellophane, we use two sheets of polaroid, as shown in Fig. 33–3. The first gives us a linearly polarized beam which we pass through the cellophane and then through the second polaroid sheet, which serves to detect any effect the cellophane may have had on the polarized light passing through it. If we first set the axes of the two polaroid sheets perpendicular to each other and remove the cellophane, no light will be transmitted through the second polaroid. If we now introduce the cellophane between the two polaroid sheets, and rotate the sheet about the beam axis, we observe that in general the cellophane makes it possible for some light to pass through the second polaroid. However, there are two orientations of the cellophane sheet, at right angles to each other, which permit no light to pass through the second polaroid. These orientations in which linearly polarized light is transmitted through the cellophane with no effect on the direction of polarization must be the directions parallel and perpendicular to the optic axis of the cellophane sheet.
We suppose that the light passes through the cellophane with two different velocities in these two different orientations, but it is transmitted without changing the direction of polarization. When the cellophane is turned halfway between these two orientations, as shown in Fig. 33–3, we see that the light transmitted through the second polaroid is bright.
It just happens that ordinary cellophane used in commercial packaging is very close to a half-wave thickness for most of the colors in white light. Such a sheet will turn the axis of linearly polarized light through 90∘ if the incident linearly polarized beam makes an angle of 45∘ with the optic axis, so that the beam emerging from the cellophane is then vibrating in the right direction to pass through the second polaroid sheet.
If we use white light in our demonstration, the cellophane sheet will be of the proper half-wave thickness only for a particular component of the white light, and the transmitted beam will have the color of this component. The color transmitted depends on the thickness of the cellophane sheet, and we can vary the effective thickness of the cellophane by tilting it so that the light passes through the cellophane at an angle, consequently through a longer path in the cellophane. As the sheet is tilted the transmitted color changes. With cellophane of different thicknesses one can construct filters that will transmit different colors. These filters have the interesting property that they transmit one color when the two polaroid sheets have their axes perpendicular, and the complementary color when the axes of the two polaroid sheets are parallel.
Another interesting application of aligned molecules is quite practical. Certain plastics are composed of very long and complicated molecules all twisted together. When the plastic is solidified very carefully, the molecules are all twisted in a mass, so that there are as many aligned in one direction as another, and so the plastic is not particularly birefringent. Usually there are strains and stresses introduced when the material is solidified, so the material is not perfectly homogeneous. However, if we apply tension to a piece of this plastic material, it is as if we were pulling a whole tangle of strings, and there will be more strings preferentially aligned parallel to the tension than in any other direction. So when a stress is applied to certain plastics, they become birefringent, and one can see the effects of the birefringence by passing polarized light through the plastic. If we examine the transmitted light through a polaroid sheet, patterns of light and dark fringes will be observed (in color, if white light is used). The patterns move as stress is applied to the sample, and by counting the fringes and seeing where most of them are, one can determine what the stress is. Engineers use this phenomenon as a means of finding the stresses in odd-shaped pieces that are difficult to calculate.
Another interesting example of a way of obtaining birefringence is by means of a liquid substance. Consider a liquid composed of long asymmetric molecules which carry a plus or minus average charge near the ends of the molecule, so that the molecule is an electric dipole. In the collisions in the liquid the molecules will ordinarily be randomly oriented, with as many molecules pointed in one direction as in another. If we apply an electric field the molecules will tend to line up, and the moment they line up the liquid becomes birefringent. With two polaroid sheets and a transparent cell containing such a polar liquid, we can devise an arrangement with the property that light is transmitted only when the electric field is applied. So, we have an electrical switch for light, which is called a Kerr cell. This effect, that an electric field can produce birefringence in certain liquids, is called the Kerr effect.
الاكثر قراءة في مواضيع عامة في علم البصريات
اخر الاخبار
اخبار العتبة العباسية المقدسة
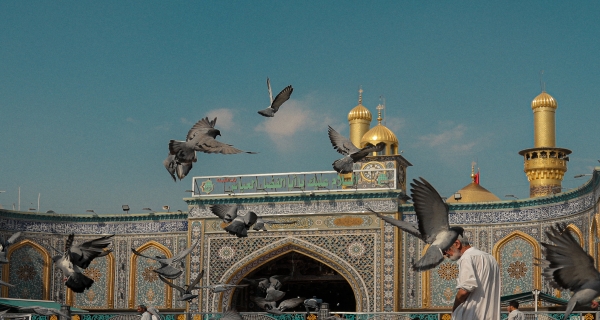
الآخبار الصحية
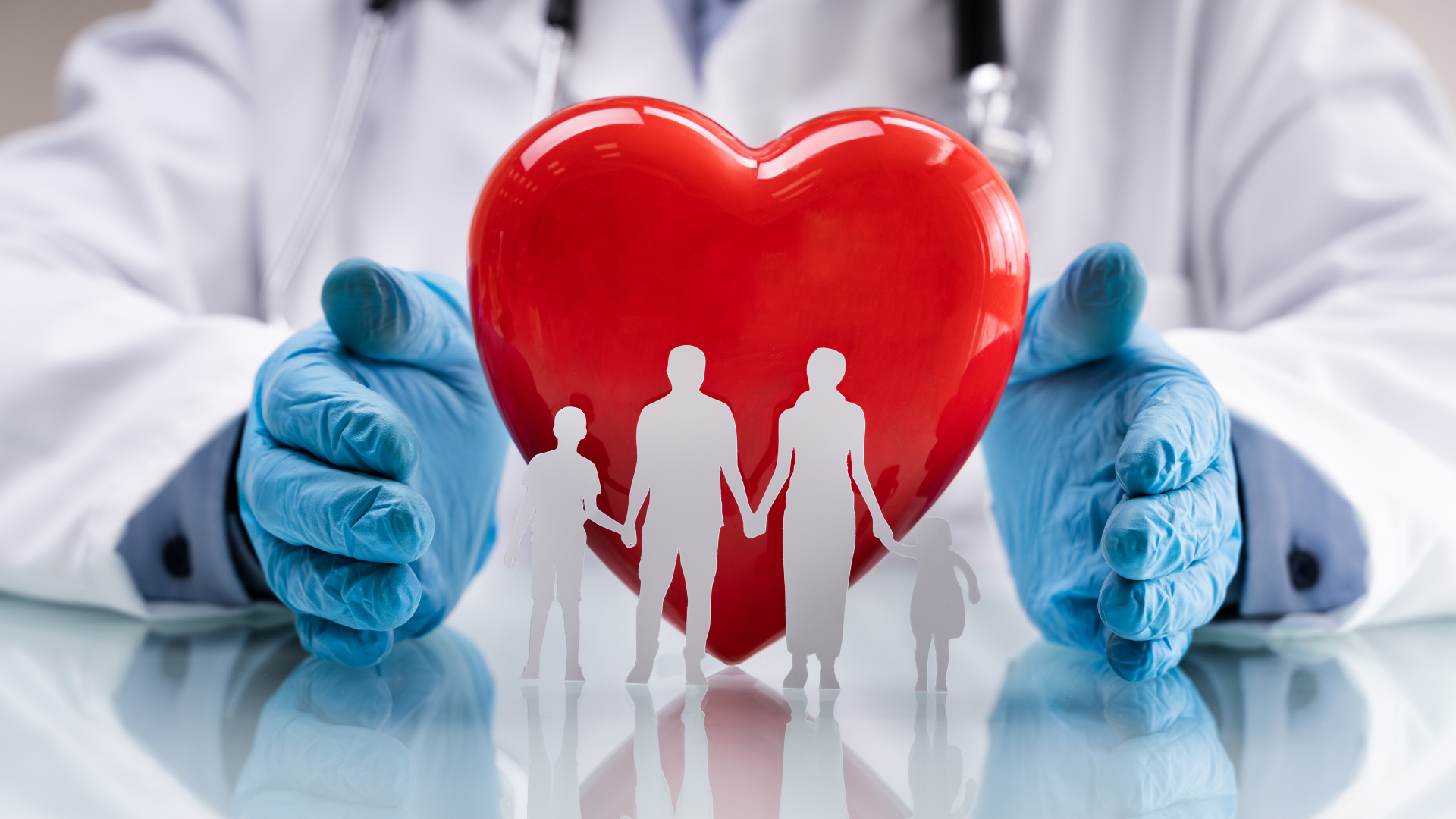