تاريخ الفيزياء
علماء الفيزياء
الفيزياء الكلاسيكية
الميكانيك
الديناميكا الحرارية
الكهربائية والمغناطيسية
الكهربائية
المغناطيسية
الكهرومغناطيسية
علم البصريات
تاريخ علم البصريات
الضوء
مواضيع عامة في علم البصريات
الصوت
الفيزياء الحديثة
النظرية النسبية
النظرية النسبية الخاصة
النظرية النسبية العامة
مواضيع عامة في النظرية النسبية
ميكانيكا الكم
الفيزياء الذرية
الفيزياء الجزيئية
الفيزياء النووية
مواضيع عامة في الفيزياء النووية
النشاط الاشعاعي
فيزياء الحالة الصلبة
الموصلات
أشباه الموصلات
العوازل
مواضيع عامة في الفيزياء الصلبة
فيزياء الجوامد
الليزر
أنواع الليزر
بعض تطبيقات الليزر
مواضيع عامة في الليزر
علم الفلك
تاريخ وعلماء علم الفلك
الثقوب السوداء
المجموعة الشمسية
الشمس
كوكب عطارد
كوكب الزهرة
كوكب الأرض
كوكب المريخ
كوكب المشتري
كوكب زحل
كوكب أورانوس
كوكب نبتون
كوكب بلوتو
القمر
كواكب ومواضيع اخرى
مواضيع عامة في علم الفلك
النجوم
البلازما
الألكترونيات
خواص المادة
الطاقة البديلة
الطاقة الشمسية
مواضيع عامة في الطاقة البديلة
المد والجزر
فيزياء الجسيمات
الفيزياء والعلوم الأخرى
الفيزياء الكيميائية
الفيزياء الرياضية
الفيزياء الحيوية
الفيزياء العامة
مواضيع عامة في الفيزياء
تجارب فيزيائية
مصطلحات وتعاريف فيزيائية
وحدات القياس الفيزيائية
طرائف الفيزياء
مواضيع اخرى
WAVELENGTH SELECTION IN MULTILINE LASERS
المؤلف:
Mark Csele
المصدر:
FUNDAMENTALS OF LIGHT SOURCES AND LASERS
الجزء والصفحة:
p166
17-3-2016
2541
WAVELENGTH SELECTION IN MULTILINE LASERS
Frequently, it is desired to operate a laser on a single wavelength. Multiple-wavelength lasers, such as the argon ion, can oscillate up to 10 discrete lines simultaneously, and dye lasers have broad gain profiles that can span over 100 nm. Single-line operation on these lasers can be achieved by selective feedback in which the optics are designed to reflect only a single wavelength. The first method of wavelength selection is to design the cavity optics themselves to be highly reflective at a single wavelength (or a set of chosen wavelengths) and trans missive (which represents a loss in the cavity) at all others. This method is commonly used with helium–neon lasers, in which it is necessary to suppress the strong infrared transition to boost output on the 632.8-nm red line. In a green helium neon laser, optics are designed carefully to reflect light centered at 543.5 nm but to transmit wavelengths such as the red. If the optics were broadband and reflected equally well in the red and green regions, the dominant red line would appear it must be suppressed to allow the green transition to oscillate. Furthermore, to oscillate, even the red helium–neon laser requires optics that suppress an infrared transition, which lasers preferentially.
Selective optics are also commonly employed in krypton-ion lasers in which the user may select which lines oscillate simply by replacing the HR with one suited for red (the single powerful Kr+ line at 647.1 nm), red yellow (allowing both the red 647.1-nm line and the yellow line at 568.2 nm to lase simultaneously), or multiline, which allows various laser lines, spanning from violet to red to oscillate. Of course, using a single-line optic (such as the red line only) will yield a much higher output power on that one line than a multiline optic would.
As an example of the usefulness of selective optics, consider a particular krypton laser in the author’s lab which produces white light. The optics are designed precisely to produce multiple wavelengths in the output beam which when added together produce white. The various major lines and relative intensities for a krypton laser are as shown in Table 1.1. This is a perfect laser for display purposes and is used extensively for laser light shows since it features output lines in every color of the spectrum, allowing full-color displays. To produce white light, the output powers of various wavelength components must be balanced. While the red line is quite powerful (producing 39% of the total power of a multiline krypton laser), and other lines, such as the yellow line, produce reasonably powerful output, krypton lacks a powerful line in the blue region of the spectrum. Also, the green line at 530.9 nm, although powerful, is a lime-green color that is undesirable for display effects.
The problem now is to design a set of optics for the laser which, for efficiency’s sake, allows only a few desired lines to oscillate and in proportions that allow the addition of these to produce white light. The reflectivity of the mirror was analyzed using a spectrophotometer and is depicted in Figure 1.1 along with krypton laser lines for reference. Owing to the fact that this is a small air-cooled laser and has low overall gain, the only lines that will lase are those where the reflectivity of the OC is considerably greater than 95% at a particular wavelength. The HR was also analyzed and has a reflectivity of greater than 99.9% throughout the range spanning from 450 to 710 nm. A quick examination of the figure shows that the reflectivity is too low at 406.7, 520.8, 530.9, and 676.4 nm to allow these krypton lines to oscillate, leaving two closely spaced blue wavelengths, one yellow wavelength, and a red wavelength. In practice, the output powers of these four lines balance, so that the beam appears white. In other words, the reflectivity of this optic at the blue wavelengths is slightly higher than at the red wavelength, so that the total output power of the blue lines is equal to that of the red line. These are minor variations in reflectivity and do not appear on the graph, given the scale involved.
Two other methods for selecting a single wavelength involve modifying the HR of the laser cavity. The addition of a prism between the plasma tube and the HR allows selection of a single line, since only one unique wavelength can make the path through the prism, reflecting off the HR and back through the prism in exactly
TABLE 1.1. Major Krypton-Ion Laser Lines and Relative Output Powers
Figure 1.1. Reflectivity of the OC for a krypton white light laser.
the same path. By changing the angle of the prism and HR relative to the laser’s axis, this arrangement allows tuning through a large range. Alternatively, the HR may be replaced by a diffraction grating which, in a similar manner, reflects only a single wavelength of light back into the plasma tube for amplification. Both methods are outlined in Figure 1.2. Practically speaking, prisms have lower losses than diffraction gratings and so are used in lower-gain (e.g., argon) lasers, where even small losses can significantly impair output power (or halt oscillation completely). Diffraction gratings, on the other hand, feature higher angular dispersions of incident wavelengths and so are easier to tune when multiple wavelengths are close together or the medium has a large continuous wavelength range, such as a dye laser.
A wavelength selector employing a prism and used to tune an argon laser is pictured in Figure 1.3. Part (a) shows the actual wavelength-selective elements, including the prism and HR, mounted together in an assembly that is locked into the rear of the laser cavity, replacing a simple HR. A precision mechanism [part (b) of the figure] allows the angle of this assembly to be tilted relative to the laser’s axis. In part (a) the metal can protecting the optical elements from dust has been removed, while in part (b) only the end of the can, with two knurled nuts, is visible in the center of the laser. The mechanism is calibrated in nanometers and allows tuning over the entire visible and near-UV range covered by the laser. While wavelength selection allows oscillation of a single wavelength, that line is relatively broad, owing to Doppler and other broadening mechanisms at play in the laser.
Figure 1.2. Wavelength selection optics.
(a)
(b)
Figure 1.3. Actual wavelength selector for an argon laser.
The cavity of the laser, itself an interferometer, divides this broadened line into a number of longitudinal modes each separated slightly in frequency. For true single-wavelength operation (with an extremely narrow spectral width), further refinement is required.
الاكثر قراءة في مواضيع عامة في الليزر
اخر الاخبار
اخبار العتبة العباسية المقدسة
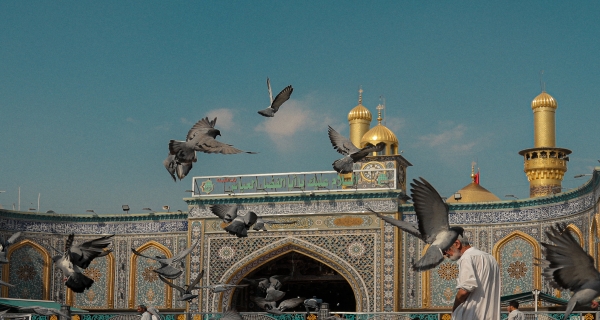
الآخبار الصحية
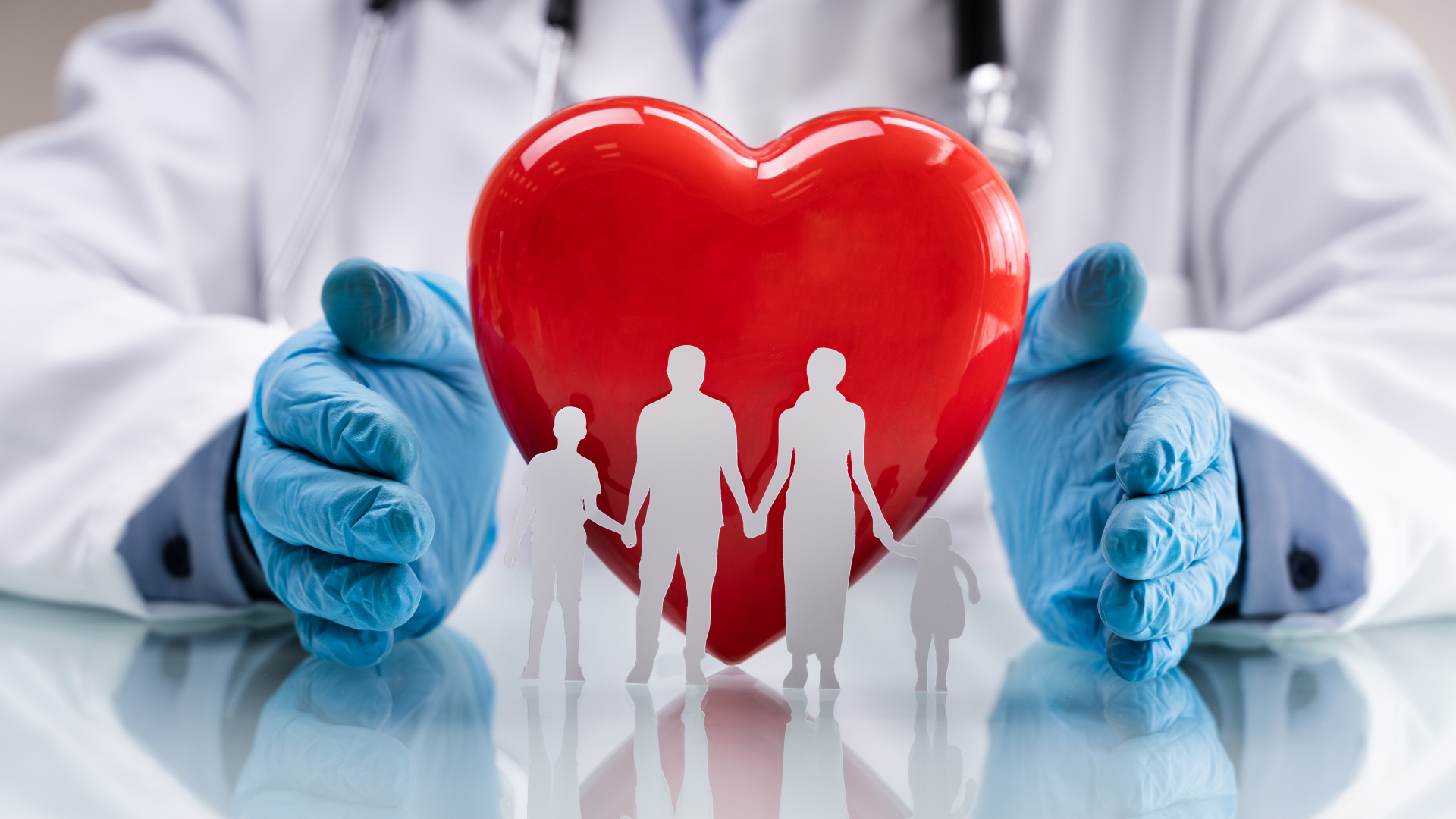