النبات
مواضيع عامة في علم النبات
الجذور - السيقان - الأوراق
النباتات الوعائية واللاوعائية
البذور (مغطاة البذور - عاريات البذور)
الطحالب
النباتات الطبية
الحيوان
مواضيع عامة في علم الحيوان
علم التشريح
التنوع الإحيائي
البايلوجيا الخلوية
الأحياء المجهرية
البكتيريا
الفطريات
الطفيليات
الفايروسات
علم الأمراض
الاورام
الامراض الوراثية
الامراض المناعية
الامراض المدارية
اضطرابات الدورة الدموية
مواضيع عامة في علم الامراض
الحشرات
التقانة الإحيائية
مواضيع عامة في التقانة الإحيائية
التقنية الحيوية المكروبية
التقنية الحيوية والميكروبات
الفعاليات الحيوية
وراثة الاحياء المجهرية
تصنيف الاحياء المجهرية
الاحياء المجهرية في الطبيعة
أيض الاجهاد
التقنية الحيوية والبيئة
التقنية الحيوية والطب
التقنية الحيوية والزراعة
التقنية الحيوية والصناعة
التقنية الحيوية والطاقة
البحار والطحالب الصغيرة
عزل البروتين
هندسة الجينات
التقنية الحياتية النانوية
مفاهيم التقنية الحيوية النانوية
التراكيب النانوية والمجاهر المستخدمة في رؤيتها
تصنيع وتخليق المواد النانوية
تطبيقات التقنية النانوية والحيوية النانوية
الرقائق والمتحسسات الحيوية
المصفوفات المجهرية وحاسوب الدنا
اللقاحات
البيئة والتلوث
علم الأجنة
اعضاء التكاثر وتشكل الاعراس
الاخصاب
التشطر
العصيبة وتشكل الجسيدات
تشكل اللواحق الجنينية
تكون المعيدة وظهور الطبقات الجنينية
مقدمة لعلم الاجنة
الأحياء الجزيئي
مواضيع عامة في الاحياء الجزيئي
علم وظائف الأعضاء
الغدد
مواضيع عامة في الغدد
الغدد الصم و هرموناتها
الجسم تحت السريري
الغدة النخامية
الغدة الكظرية
الغدة التناسلية
الغدة الدرقية والجار الدرقية
الغدة البنكرياسية
الغدة الصنوبرية
مواضيع عامة في علم وظائف الاعضاء
الخلية الحيوانية
الجهاز العصبي
أعضاء الحس
الجهاز العضلي
السوائل الجسمية
الجهاز الدوري والليمف
الجهاز التنفسي
الجهاز الهضمي
الجهاز البولي
المضادات الحيوية
مواضيع عامة في المضادات الحيوية
مضادات البكتيريا
مضادات الفطريات
مضادات الطفيليات
مضادات الفايروسات
علم الخلية
الوراثة
الأحياء العامة
المناعة
التحليلات المرضية
الكيمياء الحيوية
مواضيع متنوعة أخرى
الانزيمات
Fate Map
المؤلف:
K. Woo and S. E. Fraser
المصدر:
Development 121, 2595–2609
الجزء والصفحة:
9-5-2016
2836
Fate Map
1. Classical Fate Maps
A fate map is a graphic representation of the cells or regions within the embryo at one point during development that will develop into specific tissues and organs at subsequent developmental stages. The concept of the fate map may be one of the earliest abstract ideas in embryology, one that is implicit, for example, in the “homunculus” imagined by seventeenth century microscopists to be a miniature adult human that is “preformed” within the sperm. The concept of preformation was generally favored by scientists until the last half of the eighteenth century, when Caspar Friedrich Wolff, through observation of chick embryo development, postulated that embryos develop through a process he termed “epigenesis,” whereby unpatterned tissues of the early embryo reorganize and expand to form the tissues and organs of the adult. As the cellular basis for tissue organization came to be understood by the mid-nineteenth century, it eventually became clear that embryonic development could be characterized by four cellular phases common to all vertebrate and most invertebrate embryos: (i) formation of the single-celled, diploid zygote (or fertilized egg) through union of haploid sperm and egg; (ii) cleavage , in which the fertilized egg undergoes mitotic division without increase in size to form the multicellular blastula (mammalian: blastocyst); (iii( gastrulation, in which blastula cells rearrange and expand to form the three primary germ layers (ectoderm, mesoderm, and endoderm) that are organized into the basic body plan (concepts developed in the early nineteenth century by von Baer); and (iv) organogenesis, in which the cells within the the three primary germ layers of the embryo form the organ and tissue primordia that develop into the tissues and organs of the fetus . The fetal stage of development is distinguished from the embryonic phase in that the fetus is indeed a preformed miniature of the adult that contains all of the organ and tissue rudiments (in the right places and containing differentiated cells and stem cells( that need only to expand and mature to function as adult tissues and organs. The transition from embryo to fetus occurs at about the eighth week of human development.
In the late nineteenth and early twentieth centuries, embryologists began to take an experimental approach to the study of development. Fate maps of relatively simple embryos, such as ascidians, were initially established by directly observing the fate of blastula cells during gastrulation (1). The emergence of dyes that could be applied to living tissues without damaging them (“vital dyes” ) permitted selective staining of parts of blastula embryos and subsequent tracing of the tissues of the embryo that developed from these stained parts. Systematic application of this procedure to trace the fate of different regions of amphibian blastulas through gastrulation led to the construction of the first detailed and comprehensive fate map of a vertebrate embryo (2, 3). Subsequent work by many individuals led to the construction of fate maps for organ rudiment primordia of a variety of chordate embryos (amphioxus, fish, amphibians, birds) (4-9). These maps continue today as the primary basis for our present day understanding of morphogenetic movements (2) that blastula cells undergo during gastrulation. Nevertheless, these maps are constantly being revised and refined by applying more modern techniques. For recent examples, see fish (10, 11); birds (12-15); and amphibians (16-18).
Although fate maps, such as those described previously, give a general idea how regions of the blastula give rise to the primary organ primordia of the embryo, they do not yield precise details of the way cells within those primordia give rise to the more complex tissues and organs of the fetus and adult. These events could not be adequately followed by direct observation because of the massive expansion of cells during the embryonic phase of development. Moreover, during the ensuing cell expansion and migrations, vital dyes became diluted beyond the level of detectability. The latter problem was only partially circumvented by using microscopic carbon particles to follow cell movements and rearrangements (19, 20).
In the late 1960s, however, Nicole Le Douarin developed a powerful method for embryonic fate mapping that allowed following the mapping of embryonic tissues indefinitely, even into full adulthood. Briefly, she discovered that quail cells could be distinguished from chicken cells by a nucleolus in the quail cell that developed a brilliant magenta color when stained with the Feulgen reagent (21, 22). By transplanting homologous tissues from quail embryos into chick embryos, the fate of the transplanted quail cells could be established unequivocally by histological methods. Through broad application of this method, the development of the avian embryo has been mapped in detail Much of this newer map supports and confirms earlier work. Nevertheless, this new method brilliantly illuminated the many limitations and previous inaccuracies of earlier methods. This is particularly true for migratory mesenchyme cells within the embryo, such as the neural crest, which, as revealed by LeDouarin and co-workers, populate a previously unknown variety of tissues and organs (24-28). In addition, the embryonic development of other mobile cell populations, such as blood cell lineages (29-34) and muscle precursor cells (35-37) and many other other tissues, were extended and clarified in ways not possible previously.
2. Mammalian Embryo Fate Maps
Fate mapping in mammalian embryos progressed much more slowly because of the relative inaccessibility of these embryos within the mother's uterus. Therefore, the fate maps in most existing textbooks are derived from the avian embryo based on assumption that mammalian development is essentially identical. Because of the recent development of methods for in vitro culture of early mammalian embryos, fate mapping of early mammalian development has begun. One such fate map experiment was conducted by transplanting fragments of tissue from early mouse embryos radiolabeled with tritiated thymidine in unlabeled embryos, followed by localizing the labeled tissues by autoradiography (38). The recent development of highly fluorescent dyes that can be injected into cells has greatly facilitated fate mapping of early mouse embryo tissues (39-43) To date, the most important outcome of such studies has been to establish that existing mammalian fate maps based upon the avian model are indeed valid by and large. Therefore, it is no exaggeration to state that the methods and strategies developed by Le Douarin and the resulting detailed fate maps of the avian embryo provide the most accurate and comprehensive information garnered during the twentieth century about the cellular movements underlying vertebrate embryonic development, including mammalian and human development.
3. Embryonic Fate and Developmental Potential
At the onset of development, the single-celled zygote can form all of the cell and tissue types of the adult, and this potential is apportioned during embryogenesis. The relationship between developmental potential and developmental fate is the central question that developmental biologists have been trying to understand for more than a century [the reader is directed to a recent and excellent review and discussion by Viktor Hamburger of the early experiments in this area (44)]. Wilhelm Roux first addressed this problem experimentally in 1883 by ablating one blastomere of an amphibian embryo. He found that the surviving blastomere formed an incomplete embryo, leading him to conclude that fate and potential were apportioned in concert. Although this initial conclusion was flawed, Roux's novel and experimental approach laid the foundations for what came to be known as experimental embryology. It was not long until it was experimentally established that developmental potential is generally more widely dispersed within the early embryo than developmental fate. Thus, a specific region of the early embryo can develop into several different tissues and organs, even though its fate represents only one of those possible outcomes. As the embryo matures, the range of potentiality of these regions becomes restricted until, ultimately, potential and fate become equal, and the tissue is deemed determined (45). The mechanism(s) that control fate and potential constitute one of the central mysteries of developmental biology. The seminal experiment that established the profound depth of this mystery was reported in 1924 by Hilda Mangold, a graduate student in the laboratory of Hans Spemann. She showed that fragments of specific regions from one amphibian gastula could induce formation of a complete second embryo when transplanted into the prospective belly region of a host gastrula (46) (an English translation of this article can be found in Ref. 47). The fact that the transplanted fragment could reprogram host tissue, fated to form abdominal structures, to form head tissues, including brain, led to the “organizer” hypothesis, in which some embryonic tissues play central roles to induce and direct the development of neighboring tissues. That discovery provided the conceptual basis for virtually all developmental biology in the twentieth century. Despite the advances in the cellular and molecular analysis of development in the last two decades of this century, the mechanistic bases for organizer function, morphogenesis, and cell determination in vertebrates remain mysteries.
4. Genetic Control of Developmental Fate
By contrast, however, research into mechanisms by which genes control embryonic development has progressed most rapidly, using the fruit fly Drosophila, because of the advanced state of genetic analysis in this organism. Although a fate map of the early Drosophila embryo was available by mid-twentieth century (48), a coherent hypothesis regarding the role of specific genes in establishing the Drosophila fate map was first proposed in 1980 (49-51). This breakthrough in studying the relationship between embryonic fate maps and genetics has revolutionized the developmental biology of Drosophila. A large proportion of the genes that act developmentally in flies have vertebrate homologues that play key, but typically different, developmental roles. Thus, a definitive model for the genetic control of embryonic fate maps and pattern formation in vertebrates remains to be established.
5. The Difficult Relationship Between Fate Maps and Vertebrate Genetic Expression Patterns
Molecular probes for the expressed products of genes developed during the last half of the twentieth century have allowed superimposing fate maps and gene expression maps. The first use of such a technique can be traced back to at least 1957, when Holtzer and Detwiler used a fluorescent antimyosin antibody to localize the earliest appearance of differentiated muscle cells within the somite myotomes of chick embryos (53). This general approach expanded enormously in recent years and now includes in situ hybridization, which allows detecting the RNA products of genes. Thus the developmental expression maps of a large number of genes of both known and unknown function are now known for development of a wide range of organisms. In instances in which the patterns derived from these two types of maps coincide, it is often assumed that the molecular expression accurately reflects that of cells whose fate is known. Although this may be generally true, the assumption carries potential pitfalls of which one should be wary, at least when unaccompanied by appropriate experimental validation. Migratory cells, for example, often intermingle transiently with and are indistinguishable from nonmigratory cells. The signal from the migratory cells in such situations can be discerned if they carry an independent lineage marker, such as the quail nucleolus referred to previously, that allows distinguishing them from their nonmigratory counterparts (54). On the other hand, some gene products (particularly RNA) can appear and be degraded extremely rapidly in patterns that reflect transcriptional waves passing through specific tissues. Pourquie and co-workers discovered such transcriptional waves and distinguished them from cell migration by demonstrating discordance over time between the pattern of injected fluorescent dyes and gene expression signals (55). Finally, cells may transiently express one gene product at one period of development and then switch to expression of another. Cepko and co-workers have developed techniques in which infective retroviruses are used to follow transient expression patterns of individual cells and their progeny through complex developmental pathways (56-59). These examples serve only to illustrate that gene expression patterns can only be assumed to reflect cell lineage or fate maps until experimentally verified by independent means.
6. Transgenic Strategies for Fate Mapping
Molecular techniques are now being used to map developmental fate and potential and also to manipulate them experimentally. Retroviruses are widely used to deliver bioactive genes to influence development positively or negatively through the expression of exogenous gene products. The emergence of methods for creating germ-line transgenic mice has led to a variety of new and imaginative approaches to analyzing of the relationship between cell fate and gene expression in mammalian development. Buckingham and co-workers have “knocked-in” a beta-galactosidase marker gene into a gene locus, which that controls the onset of myogenesis, to locate newly “born” muscle precursor cells in the mouse embryo (62, 63). Recently, transgenes that must undergo recombination signals to be expressed in a cell- or tissue-specific fashion have been used by Nicolas and co-workers to track cell lineage progression in early mouse development (64-66). It is important to keep in mind that, because each of these techniques depends on cell- or tissue-specific gene regulation (which is itself only beginning to be understood), each is subject to the caveats alluded to previously for the relationship between cell fate and gene expression. Nevertheless, the power of these new techniques augers well for the future analysis of mechanisms underlying the apportionment of cell fate and potential during embryonic development.
References
1. E. G. Conklin (1905) J. Acad. Nat. Sci. (Philadelphia) 2, 13.
2. W. Vogt (1929) Roux’ Arch. 120, 385–706.
3. W. Vogt (1925) Roux’ Arch. 106, 542–610.
4. R. Wetzel (1929) Roux’ Arch. 119, 118–321.
5. E. G. Conklin (1932) J. Morphol. 54, 69–118.
6. J. M. Oppenheimer (1936) J. Exp. Zool. 72, 409–437.
7. J. Pasteels (1940) Biol. Rev. 15, 59–106.
8. D. Rudnick (1943) Q. Rev. Biol. 19, 187.
9. J. M. Oppenheimer (1947) Q. Rev. Biol. 22, 105–118.
10. K. Woo and S. E. Fraser (1995) Development 121, 2595–2609.
11. J. Shih and S. E. Fraser (1995) Development 121, 2755–2765.
12. H. Eyal-Giladi and S. Kochav, (1976) Dev. Biol. 49, 321–337.
13. M. A. J. Selleck and C. D. Stern (1991) Development 112, 615–626.
14. Y. Hatada and C. D. Stern (1994) Development 120, 2879–2890.
15. G. C. Schoenwolf, V. Garcia-Martinez, and M. S. Dias (1992) Developmental Dynamics 193, 235-248.
16. S. B. Minsuk and r. R. E. Kelle (1996) Developmental Biol. 174, 92–103.
17. T. Elul, M. A. Koehl, and R. Keller (1997) Developmental Biol. 191, 243–258.
18. R. Harland and J. Gerhart (1997) Annu. Rev. Cell Developmental Biol. 13, 611–667.
19. N. T. Spratt Jr. (1946) J. Exp. Zool. 103, 259–304.
20. R. C. Fraser (1954) J. Exp. Zool. 126, 349–399.
21. N. M. Le Douarin (1969) Bull. Biol. Fr. Belg. 103, 435–452.
22. N. Le Douarin (1973) Exp. Cell Res. 77, 459–468.
23. N. M. Le Douarin and M. A. Teillet, eds. (1984) Chimeras in Developmental Biology, Academic Press, London, San Diego.
24. N. M. Le Douarin and M. A. Teillet (1973) J. Embryol. Exp. Morphol. 30(1), 31–48.
25. N. M. Le Douarin (1975) Birth Defects Original Article Series, 11(7), 19–50.
26. J. Fontaine, C. Le Lievre, and N. M. Le Douarin (1977) Gen. Comp. Endocrinol. 33(3), 394–404.
27. C. S. Le Lievre and N. M. Le Douarin (1975) J. Embryol. Exp. Morphol. 34(1), 125–154.
28. J. M. Polak et al. (1974) Histochemistry 40(3), 209–214.
29. E. Houssaint, M. Belo, and N. M. Le Douarin (1976) Developmental Biol. 53(2), 250–264.
30. F. V. Jotereau, E. Houssaint, and N. M. Le Douarin (1980) Eur. J. Immunol . 10(8), 620–627.
31. N. M. Le Douarin and F. V. Jotereau (1975) J. Exp. Med. 142(1), 17–40.
32. N. M. Le Douarin et al. (1975) Proc. Nat. Acad. Sci. USA 72(7), 2701–2705.
33. N. M. Le Douarin, E. Houssaint, and F. Jotereau (1977) Adv. Exp. Med. Biol. 88, 29–37.
34. N. M. Le Douarin, F. Dieterlen-Lievre, and P. D. Oliver (1984) Am. J. Anat. 170(3), 261–299.
35. B. Christ, H. J. Jacob, and M. Jacob (1974) Experientia 30(12), 1446–1449.
36. B. Christ, H. J. Jacob, and M. Jacob (1977) Anat. Embryol. 150(2), 171–186.
37. A. Chevallier, M. Kieny, and A. Mauger (1977) J. Embryol. Exp. Morphol. 41, 245–258.
38. P. P. L. Tam and R. S. P. Beddington (1987) Development 99, 109–126.
39. K. A. Lawson, J. J. Meneses, and R. A. Pederson (1991) Development 113, 891–911.
40. M. Parameswaran and P. P. L. Tam (1995) Dev. Genet. 17, 16–28.
41.P. P. L. Tam and S. X. Zhou (1996) Developmental Biol . 178, 124–132.
42. P. P. L. Tam and R. R. Behringer (1997) Mech. Dev. 68, 3–25.
43. P. P. L. Tam et al. (1997) Development 124, 1631–1642.
44. V. Hamburger (1988) The Heritage of Experimental Embryology: Hans Spemann and the Organizer, Monographs on the History and Philosophy of Biology (R. B. R. Burian Jr., R. Lewontin, and J. M. Smith, eds.), Oxford University Press, New York and Oxford.
45. H. Spemann (1938) Embryonic Development and Induction Yale University Press, New Haven, CT.
46. H. Spemann and H. Mangold (1924) Roux’ Archiv. 100, 599–638.
47. B. H. Willier and J. Oppenheimer (1974) Foundations of Experimental Embryology, 2nd ed., Hafner, New York.
48. D. F. Poulson (1950) Biology of Drosophila (M. Demerec, ed.), Hafner, New York, pp. 168–270.
49. C. Nusslein-Volhard and E. Wieschaus (1980) Nature 287, 795–801.
50. S. Roth, D. Stein, and C. Nusslein-Volhard (1989) Cell 59, 1189–1202.
51. C. Nusslein-Volhard and S. Roth (1989) Ciba Foundation Symposium 144, 37–55; discussion 98, 55-64.
52. C. Nusslein-Volhard (1979) In Determinants of Spatial Organization (S. S. a. I. R. Konigsberg, ed.), New York, Academic Press, pp. 185–211. 53. H. Holtzer, J. M. Marshall, and H. Finck (1957) J. Biophys. Biochem. Cytol. 3, 705–729.
54. B. A. Williams and C. P. Ordahl (1994) Development 120, 785–796.
55. I. Palmeirin et al. (1997) Cell 91, 639–648.
56. D. L. Turner, E. Y. Snyder, and C. L. Cepko (1990) Neuron 4(6), 833–845.
57. J. Price, D. Turner, and C. Cepko (1987) Proc. Natl. Acad. Sci. USA 84(1), 156–160.
58. C. Cepko et al. (1995) Methods Enzymol. 254, 387–419.
59. J. A. Golden, S. C. Fields-Berry, and C. L. Cepko (1995) Proc. Natl. Acad. Sci. USA 92(12), 5704–5708 .
60. C. P. Austin et al. (1995) Development 121(11), 3637–3650.
61. D. J. Goff and C. J. Tabin (1997) Development 124, 627–636.
62.S. Tajbakhsh et al. (1996) Developmental Dynamics 206, 291–300.
63. S. Tajbakhsh, D. Rocancourt, and M. Buckingham (1996) Nature 384, 266–270.
64. J. F. Nicolas, L. Mathis, and C. Bonnerot (1996) Development 122, 2933–2946.
65. L. Mathis et al. (1997) Development 124, 4089–4104.
66. D. L. Zinyk et al. (1998) Curr. Biol. 8, 665–668.
الاكثر قراءة في مواضيع عامة في الاحياء الجزيئي
اخر الاخبار
اخبار العتبة العباسية المقدسة
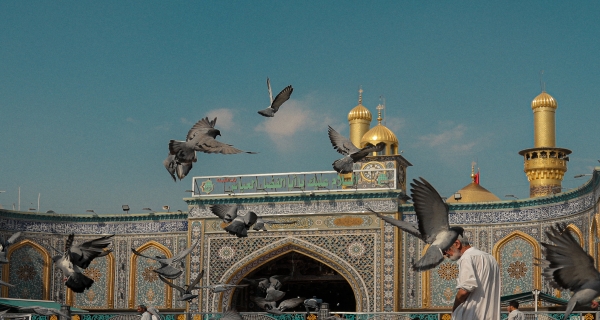
الآخبار الصحية
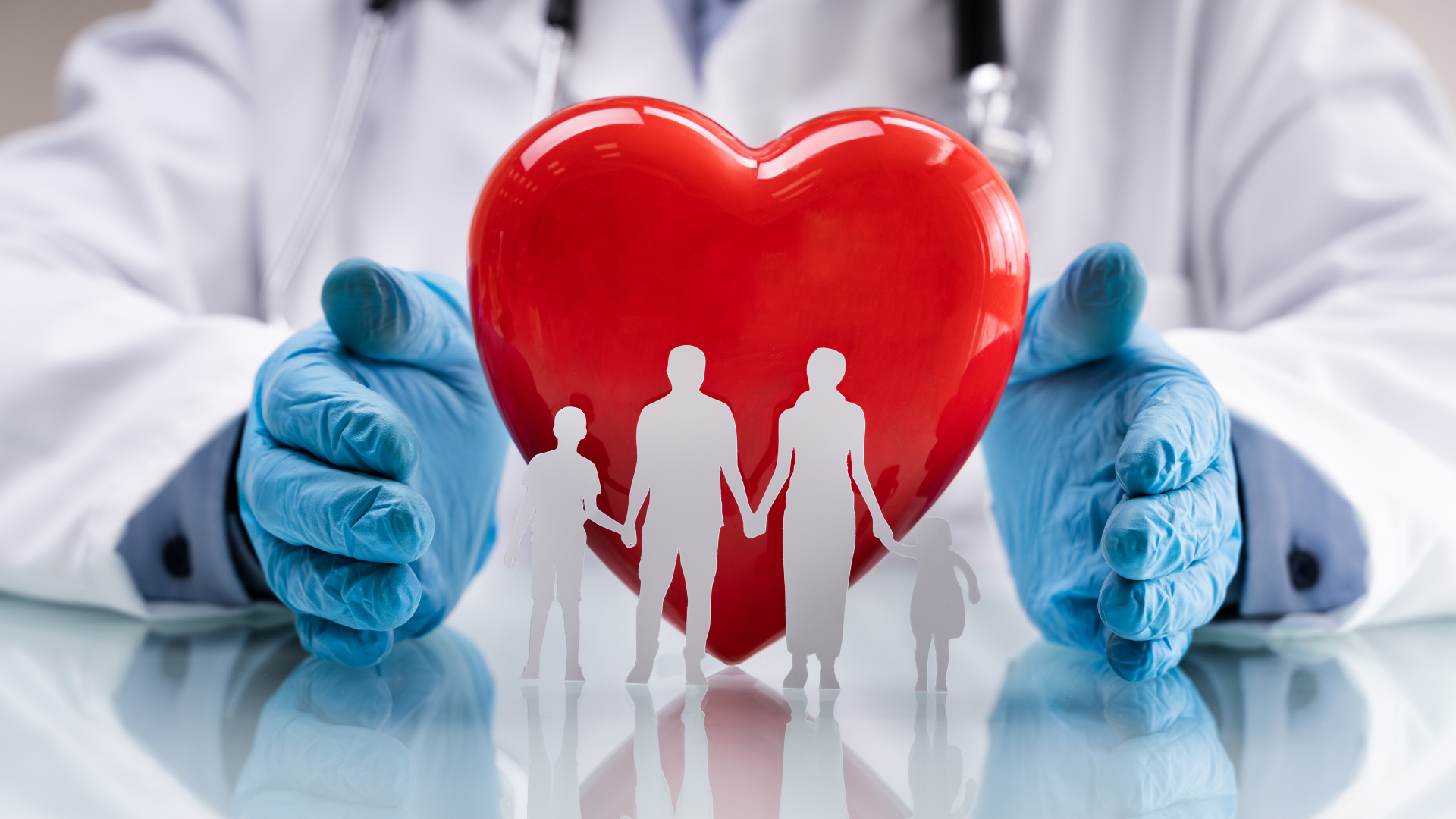